Codon Translation: Mechanisms and Implications
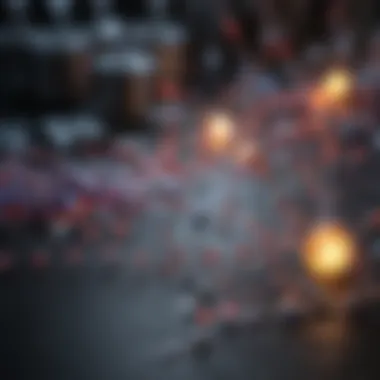
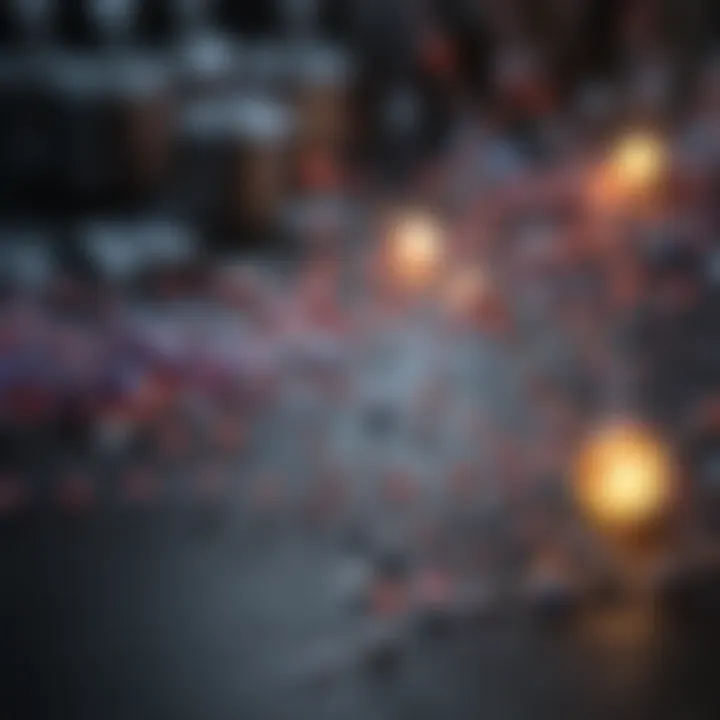
Intro
Codon translation is fundamental to the field of molecular biology. Proteins are the workhorses of the cell, and their synthesis relies on a set of rules defined by the genetic code. This process involves translating the sequence of codons in mRNA into an amino acid sequence, ultimately forming proteins. The focus will be on the mechanisms involved in codon translation, the components necessary for this process, and its implications within larger biological systems.
Understanding codon translation is not just an academic exercise; it has significant implications for genetics, biotechnology, and medicine. By exploring how errors in translation can lead to diseases, we can appreciate the importance of precision in this biological machinery. Thus, a detailed examination of codon translation connects molecular biology with real-world applications, making this an essential area of study for students, researchers, educators, and professionals.
Research Overview
Key Findings
The exploration of codon translation has revealed several critical insights:
- The role of ribosomes as the site for protein synthesis.
- The importance of tRNA in matching codons with the appropriate amino acids.
- The impact of various factors, including initiation, elongation, and termination stages of translation.
- How mutations and other errors can affect protein function, potentially leading to diseases.
Study Methodology
Research in codon translation typically integrates biochemical experiments, molecular biology techniques, and computational modeling. For example, scientists often utilize:
- Clone sequencing for understanding genetic elements involved in translation.
- In vitro translation systems to investigate the efficiency and fidelity of translation.
- Bioinformatics tools to predict the impact of mutations.
Such methodologies have provided insights into translation accuracy and efficiency, emphasizing the complexity of this process.
Background and Context
Historical Background
The concept of codon translation emerged prominently in the mid-20th century with the elucidation of the genetic code. Early researchers like Marshall Nirenberg and Har Gobind Khorana contributed immense knowledge, paving the way for understanding how sequences of nucleotides are converted to amino acids. This historical context is key to grasping the evolution of research in this area.
Current Trends in the Field
Presently, research on codon translation is vibrant and multifaceted. Advancements in technologies such as CRISPR and RNA sequencing have opened new avenues for investigation. These tools not only enhance our understanding of translation mechanisms but also allow for the exploration of how translation impacts gene regulation. Current trends also focus on the role of non-canonical codons and the impact of environmental factors on translation fidelity, indicating the field is progressing rapidly.
"Codon translation is essential for protein synthesis and lays the foundation of life as we know it."
In summary, codon translation serves as a gateway into the intricate interplay between genetics and protein function. Each discovery adds layers to our understanding and augments the potential for future research, particularly in fields such as synthetic biology and gene therapy.
Prolusion to Codon Translation
Codon translation serves as a pivotal process in molecular biology, primarily responsible for the conversion of genetic information into functional proteins. Understanding codon translation is essential for students, researchers, and educators involved in genetics, biochemistry, and biotechnology. This section aims to outline its critical importance, explore its foundational definitions, and consider its historical context, giving readers insight into why codon translation remains a focal point in current biological research.
Definition and Importance
Codon translation refers to the process wherein the sequence of nucleotide triplets within messenger RNA (mRNA) is converted into a sequence of amino acids to form proteins. Each triplet nucleotide forms a codon, which corresponds to specific amino acids or signals during protein synthesis. The intricate nature of this process underscores its significance.
The importance of codon translation is multifaceted. Firstly, it is fundamental to understanding how genetic information directs cellular functions. Proteins perform a variety of roles in biological systems, ranging from acting as enzymes to providing structural support. Research indicates that errors in codon translation can lead to malfunctioning proteins, resulting in diseases such as cystic fibrosis or sickle cell anemia. Consequently, comprehension of this process offers insights into not only basic biology but also clinical implications where understanding mutations can lead to novel therapeutic approaches.
Historical Perspective
The study of codon translation is rooted in a rich history that stretches back to early deciphering of the genetic code in the mid-20th century. In 1961, Marshall Nirenberg and Heinrich Matthei advanced our understanding by demonstrating that specific codons correspond to specific amino acids. This was a groundbreaking achievement in molecular biology, converting abstract genetic sequences into tangible protein syntesis. Their work laid the groundwork for future discoveries, illustrating the universality of the genetic code among different organisms.
The historical perspective is valuable, as it demonstrates how far molecular biology has come. Codon translation is now understood not only as a biological mechanism but also as a key component in the fields such as synthetic biology, where engineered proteins play a role in biotechnology applications. The breakthroughs in understanding codon translation have significantly shaped modern genetics research, establishing a foundation upon which current studies continue to build.
Overall, understanding codon translation not only enriches our knowledge of genetic mechanisms but also opens avenues for advancements in gene therapy, biotechnology, and synthetic biology.
The Genetic Code
The genetic code represents the fundamental system through which information encoded in DNA is translated into functional proteins. Understanding this code is paramount in molecular biology as it provides insights into how genetic information dictates cellular functions and influences phenotype. The genetic code consists of codons, which are sequences of three nucleotides that correspond to specific amino acids or serve as signals during the translation process. This framework facilitates the assembly of proteins, making the understanding of the genetic code not just important, but essential for studying life at the molecular level.
The implications of the genetic code extend beyond mere protein synthesis. The arrangement and combination of codons can lead to variations in protein structures and functions, impacting everything from cellular regulation to evolutionary processes. By defining what amino acids correspond to which codons, the genetic code serves as a universal language for biology, bridging many aspects of biochemistry and genetic engineering.
Nature of Codons
Codons are integral components of the genetic code. In essence, these three-nucleotide sequences are responsible for translating the genetic information contained within mRNA into a specific sequence of amino acids in a protein. Each of the 64 possible codons specifies an amino acid or a termination signal during translation. This association of codons with amino acids showcases the precision of molecular biology. For instance, the codon AUG not only signifies the amino acid methionine but also serves as the start signal for translation, thus beginning the synthesis of many proteins.
Moreover, the redundancy within the genetic code is noteworthy. This means that multiple codons can encode the same amino acid. For example, while leucine can be signaled by UUA, UUG, CUU, CUC, CUA, and CUG, this redundancy exhibits resilience against mutations, helping maintain functional protein synthesis despite potential changes at the nucleotide level.
Codon Classification
Codons can be classified based on their functions and properties. The primary classifications include:
- Sense Codons: These codons specify amino acids. For example, UUU is the codon for phenylalanine.
- Nonsense Codons: Also known as stop codons, these signal the termination of protein synthesis. There are three nonsense codons: UAA, UAG, and UGA.
- Start Codon: The start codon marks where translation begins. As mentioned, AUG acts both as a start codon and specifies methionine.
Moreover, codons can further be categorized according to their roles in specific organisms. For instance, the mitochondrial code differs slightly from the universal genetic code, leading to variations in how certain amino acids are encoded. This classification is helpful for researchers studying mutations and their impacts on protein function, allowing for a more nuanced understanding of genetic phenomena.

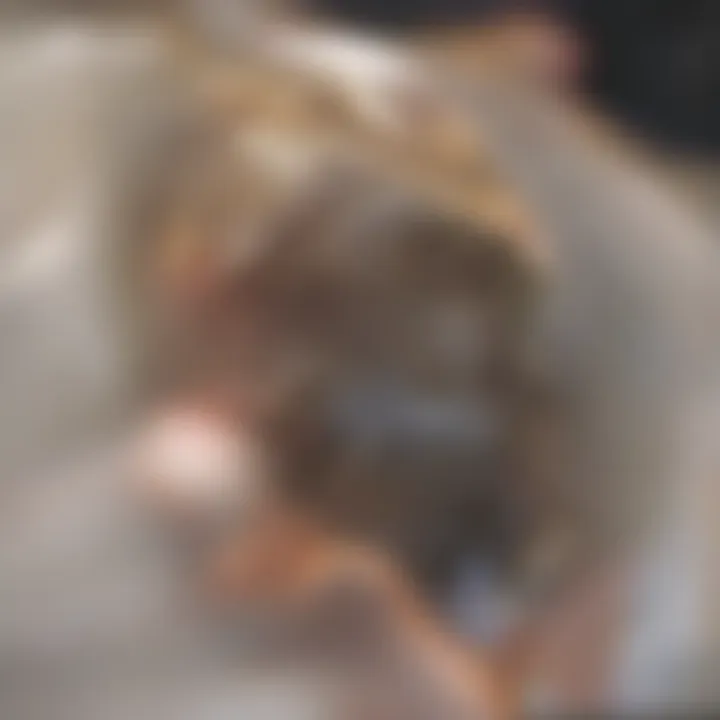
"The genetic code is not merely a blueprint for protein construction; it represents a complex interplay of biochemical interaction that dictates life processes."
Understanding codons and their classifications enhances the comprehension of protein synthesis, evolutionary biology, and genetic engineering. The exploration of codons thus serves as a foundational element in any inquiry into molecular biology.
Mechanism of Translation
Understanding the mechanism of translation is vital to comprehending how genetic information is converted into functional proteins. This process involves several intricate stages, each playing a significant role in ensuring that proteins are synthesized accurately and efficiently. Translation occurs in several key phases: initiation, elongation, and termination. Each phase must work seamlessly to maintain the fidelity and speed of protein synthesis, impacting everything from cellular function to organismal development.
Initiation Phase
The initiation phase of translation is where the machinery for protein synthesis assembles at the mRNA. This phase typically starts when the small subunit of the ribosome binds to the mRNA molecule. A crucial element of initiation involves the recognition of the start codon, usually AUG, which codes for Methionine. Initiation factors help facilitate this binding, ensuring that the ribosome is positioned correctly.
During this phase, the initiator tRNA carrying Methionine also binds to the start codon, bringing the first amino acid into place. Following this, the large subunit of the ribosome joins to form a functional ribosome. This assembly not only marks the transition to the elongation phase but is also critical for the proper regulation of the entire translation process.
Elongation Phase
Once the initiation phase is complete, translation proceeds to the elongation phase. This phase is characterized by the sequential addition of amino acids to form a polypeptide chain. Here, the ribosome moves along the mRNA, and each codon is read one at a time.
The elongation factor proteins play an important role during this phase. Each new tRNA molecule, with its corresponding amino acid, enters the ribosome's A site. Once an amino acid is added, the polypeptide chain shifts from the P site to the A site, allowing the ribosome to move forwards. This process continues until a stop codon is encountered. This precise mechanism is critical for accurate protein synthesis, as any errors can lead to dysfunctional proteins.
Termination Phase
The termination phase occurs when the ribosome reaches a stop codon on the mRNA. Notably, stop codons do not code for any amino acids, which distinguishes them from other codons. Instead, release factors bind to the ribosome, prompting the disassembly of the ribosomal subunits and the release of the newly synthesized polypeptide chain. This phase is crucial, as it effectively ends the translation process and leads to protein release.
In summary, each of these phases in the mechanism of translation is essential for the accurate synthesis of proteins. Understanding these phases not only provides insight into molecular biology but also lays the groundwork for advancements in fields like genetics and biotechnology.
Proper understanding of these mechanisms is pivotal for applications in drug discovery, genetic engineering, and synthetic biology.
Maintaining integrity during translation is crucial in ensuring that gene expression is accurately represented in functional proteins. Errors in any phase, from initiation to termination, can have significant implications for cellular function and health.
Key Players in Translation
Understanding the key players in translation is vital in grasping how genetic information is converted into functional proteins. These components each have distinct roles that interconnect systematically to ensure accuracy and efficiency in the translation process. This section delves into three primary players: mRNA, tRNA, and ribosomes, highlighting their respective functions and significance in protein synthesis.
Role of mRNA
Messenger RNA, or mRNA, is essential as it serves as the blueprint for protein synthesis. This molecule is transcribed from DNA and carries the genetic code necessary for constructing proteins. The importance of mRNA cannot be overstated; without it, the translation process would simply not occur. It contains codons, sequences of three nucleotides that specify which amino acids will be added to the growing protein chain.
When mRNA is synthesized, it undergoes several modifications, including capping and polyadenylation, which enhance its stability and translational efficiency. These modifications also protect mRNA from degradation, allowing it to persist long enough for the translation machinery to recognize and translate it. Once it reaches the ribosome, ribosomal complexes read the codons in the mRNA, guiding the addition of the correct tRNA molecules that correspond to those codons.
Key Functions of mRNA:
- Blueprint for Proteins: Provides the sequence that determines the amino acid order.
- Transcription Product: Formed from the DNA template, initiating the translation process.
- Modification: Enhances stability and regulatory connections, essential for efficient translation.
tRNA Functionality
Transfer RNA, or tRNA, plays a crucial role in protein translation by bridging mRNA codons and their corresponding amino acids. A tRNA molecule has a distinct feature—a site known as the anticodon, which is made up of three nucleotides that are complementary to the mRNA's codons. This complementarity is key, ensuring that the correct amino acid is incorporated into the growing polypeptide chain based on the mRNA sequence.
The functionality of tRNA also extends to amino acid attachment. Each tRNA picks up a specific amino acid from the pool in the cytoplasm, facilitated by enzymes known as aminoacyl-tRNA synthetases. This ensures that each tRNA is linked to its respective amino acid before participating in translation.
Significant Attributes of tRNA:
- Codon-Anticodon Interaction: Ensures accuracy in amino acid delivery.
- Amino Acid Activation: tRNA must be charged with the proper amino acid to function effectively.
- Adaptability: Various tRNA types exist to accommodate the diversity of codons.
Ribosome Structure and Function
Ribosomes are the molecular machines that orchestrate the translation process. Their multifaceted structure consists of ribosomal RNA (rRNA) and proteins, forming two subunits: the large subunit and the small subunit. The coordination of these subunits enables ribosomes to carry out their central role in synthesizing proteins.
During translation, ribosomes facilitate the binding of mRNA and tRNA. The small subunit binds mRNA first, while the large subunit accommodates tRNA. Ribosomes have distinct sites—A (aminoacyl), P (peptidyl), and E (exit)—which manage the flow of tRNA and the formation of peptide bonds between amino acids. This helps construct proteins efficiently and accurately.
Key Roles of Ribosomes:
- Site of Protein Synthesis: Hub where mRNA and tRNA interactions occur.
- Catalytic Activity: Facilitates peptide bond formation, combining amino acids into polypeptides.
- Multiple Sites: A, P, and E sites organize the timely addition of tRNAs and peptide growth.
Understanding these key players—mRNA, tRNA, and ribosomes—sheds light on the complexity and precision of the translation process, reinforcing the foundational aspects of genetic expression and metabolic functionalities.
Post-Translational Modifications
Post-translational modifications (PTMs) play a significant role in dictating the function, structure, and stability of proteins synthesized during the codon translation process. Once a polypeptide chain is formed, it does not exist as a functionally active protein until various molecular modifications occur. These processes can have profound implications in molecular biology, impacting everything from enzyme activity to cellular signaling pathways.
The importance of PTMs can be understood through the lens of protein diversity and function. While the genetic code provides a foundation for protein synthesis, it is the post-translational modifications that shape the final functional protein. Modifications are also essential for regulating protein interactions and can determine cellular localization. Understanding these modifications is crucial for grasping their biological significance and potential applications in biotechnology and medicine.
Types of Modifications
Post-translational modifications encompass a wide variety of chemical changes that occur after protein synthesis. These modifications can be broadly classified into several categories:
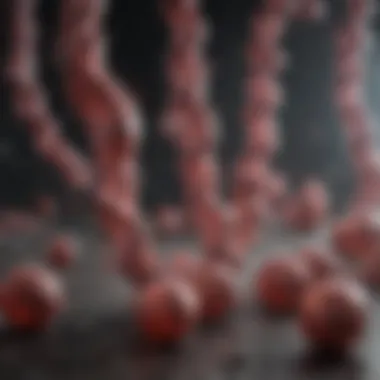
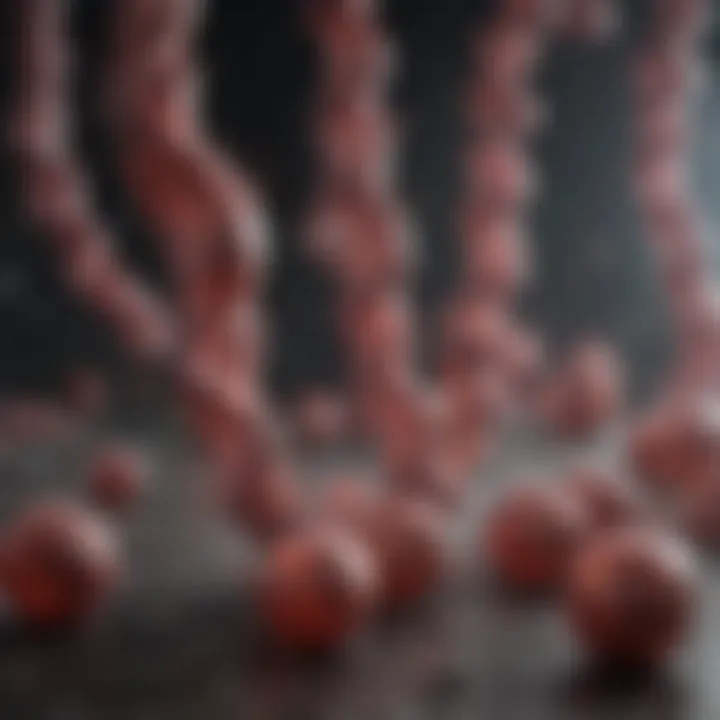
- Phosphorylation: The addition of phosphate groups, typically on serine, threonine, or tyrosine residues, is vital for regulating enzyme activity and signaling pathways.
- Glycosylation: This involves the addition of carbohydrate moieties, influencing protein stability, recognition, and localization.
- Ubiquitination: The addition of ubiquitin proteins marks proteins for degradation by the proteasome, playing a key role in protein regulation and quality control.
- Acetylation: This modification impacts the interaction of proteins with DNA and can influence gene expression.
- Methylation: Addition of methyl groups can affect protein function and interactions, particularly in histones, which are crucial for DNA packaging.
Each modification type serves specific functions and can markedly alter a protein's characteristics. The dynamic nature of these modifications highlights their adaptability in responding to cellular needs.
Impact on Protein Function
Post-translational modifications have a direct and profound impact on how proteins function within a cellular context. They are not merely finishing touches; rather, they are integrally tied to a protein's behavior and role within biological systems.
For example, phosphorylation can activate or deactivate enzymes, leading to changes in metabolic pathways. Glycosylation improves protein solubility and stability, thus playing a vital role in signaling processes. Ubiquitination ensures proper protein turnover, preventing the accumulation of damaged or redundant proteins, which is essential for maintaining cellular health.
Moreover, the implications of these modifications extend to human health. Abnormal PTMs are often linked to diseases such as cancer, diabetes, and neurodegenerative disorders. By studying these modifications, scientists can develop therapeutic approaches that target specific pathways to mitigate disease effects.
In summary, post-translational modifications are indispensable in shaping the functional landscape of proteins. The interplay between different modifications adds a layer of complexity that is crucial for effective cellular operation, thus asserting their importance in molecular biology and biotechnology.
Quality Control in Translation
Quality control in translation is essential for ensuring accuracy and efficiency in protein synthesis. The process of translating mRNA into proteins is complex, involving numerous molecular players. Any errors in this process can lead to malfunctioning proteins, which could disrupt cellular functions and lead to disease. Therefore, understanding the mechanisms of quality control provides insights into how cells maintain fidelity during translation and avoid errors that could have significant implications in molecular biology.
Fidelity Mechanisms
Fidelity mechanisms are critical in maintaining the precision of codon translation. These mechanisms operate at multiple levels to reduce the likelihood of errors occurring during protein synthesis. For example, tRNA molecules are charged with specific amino acids by enzymes called aminoacyl-tRNA synthetases. These enzymes have a high specificity for their substrates, which decreases mischarging of tRNA.
Additionally, the ribosome plays an important role in ensuring fidelity. It carefully evaluates the pairing of codons and anticodons during translation. Only when there is a stable interaction do the ribosomal subunits catalyze peptide bond formation. The GTPase activity of elongation factors also contributes to this fidelity. It helps in the proofreading of incorrect tRNA-amino acid pairs by promoting the release of mischarged tRNA before peptide synthesis occurs.
"The quality control mechanisms are effectively checkpoints that mitigate potential translation errors, thereby safeguarding the integrity of protein synthesis."
Error Detection and Repair Processes
Despite the robustness of fidelity mechanisms, errors can still occur during translation. Cells have developed sophisticated error detection and repair processes to address these inaccuracies. One such mechanism involves the use of GTPase-associated factors that inspect the ribosome and its components during translation.
When an incorrect tRNA is bound, these factors can induce a conformational change in the ribosome, leading to the release of the mismatched tRNA. This action highlights the dynamic nature of the ribosome as a quality control center. In addition, post-translational modifications are sometimes employed to mark defective proteins for degradation, which prevents the accumulation of non-functional or harmful proteins.
Overall, the combination of fidelity mechanisms and error detection processes underscores the importance of quality control in translation. Ensuring the accurate production of proteins is vital for the proper functioning of cellular systems. This topic not only emphasizes the sophistication of molecular biology but also underscores the challenges that must be overcome to achieve high fidelity in protein synthesis.
Translational Regulation
Translational regulation is a critical component of gene expression, controlling the level and timing of protein synthesis. This regulation ensures that cells can respond promptly to various internal and external stimuli. It also plays a key role in cellular differentiation and adaptation. By carefully modulating the translation process, cells maintain metabolic balance, optimize resource allocation, and adapt to changing environments.
In this section, the mechanisms of regulation and their impact on gene expression will be examined in detail. Understanding translational regulation has significant implications for biotechnology, medicine, and research in molecular biology.
Mechanisms of Regulation
Several mechanisms govern translational regulation. These mechanisms can affect all stages of translation, from initiation to termination. Some well-studied methods include:
- mRNA Stability: The lifespan of mRNA can impact how long it is available for translation. Stabilization of mRNA often enhances protein synthesis, while degradation reduces translation efficiency.
- Regulatory Proteins: Specific proteins can bind to mRNA sequences. These proteins can either promote or inhibit translation by influencing ribosome recruitment or making the mRNA structure less accessible.
- Small Non-Coding RNAs: Molecules such as microRNAs can bind to mRNAs, leading to translational repression or degradation, thus regulating the expression of target genes.
- Upstream Open Reading Frames (uORFs): These are sequences found in the mRNA that can act as signal points for translation initiation. The presence of uORFs can limit the translation of the main coding sequence under certain conditions.
These approaches allow cells to finely tune protein levels in response to various signals, contributing to dynamic cellular functions.
Impact on Gene Expression
The impact of translational regulation on gene expression is profound. By controlling when and how effectively a gene is expressed, cells can tailor protein synthesis to meet their requirements. Some important aspects include:
- Adaptive Responses: Cells can quickly synthesize proteins essential for survival, especially when responding to stress or changes in nutrient availability. This adaptability is vital in fluctuating environments.
- Cellular Differentiation: During development, translational regulation underlies the differentiation of cells into specialized types. This process often involves selective translation of specific mRNAs.
- Disease Implication: Dysregulation of translation can contribute to various diseases, including cancer and neurodegenerative disorders. For instance, the misregulation of regulatory proteins can lead to abnormal protein levels, affecting cellular homeostasis.
Overall, understanding translational regulation provides insights into fundamental biological processes and potential translational applications in fields like synthetic biology and genetic engineering.
"Understanding the mechanisms of translational regulation can lead to innovative solutions in disease therapy and biotechnology."
Mutations and Their Effects on Translation
Mutations play a critical role in the field of molecular biology, especially within the context of translation. This section aims to shed light on the various types of mutations that can occur and their subsequent effects on protein synthesis. Understanding mutations is essential, as they can result in alterations in protein function, which can significantly impact cellular processes and organismal phenotypes.
Types of Mutations
Mutations can be categorized into several types, each with distinct characteristics and implications:
- Point Mutations: These involve a change in a single nucleotide. For instance, a change of adenine (A) to guanine (G) is a point mutation. Point mutations can be classified further into three categories:
- Insertions and Deletions: These mutations involve adding or removing nucleotides from the DNA sequence. Even a single nucleotide insertion or deletion can cause a frameshift mutation, which alters the reading frame of the genetic code, potentially leading to completely different and often nonfunctional proteins.
- Duplication Mutations: In these instances, a section of DNA is duplicated, which can lead to an increase in gene expression or protein production. This can have various effects depending on the context in which they occur.
- Inversions: This occurs when a segment of DNA is reversed within the chromosome. It can disrupt the function of genes in the affected area.
- Silent Mutations: These do not cause any change in the protein sequence because they occur in non-coding regions or result in the same amino acid being produced.
- Missense Mutations: These lead to the substitution of one amino acid for another in a protein, which can affect the protein's function.
- Nonsense Mutations: These create a premature stop codon, resulting in a truncated protein that is usually nonfunctional.
Consequences for Protein Synthesis
Mutations can have profound consequences for protein synthesis. The impacts vary significantly based on the mutation type and where it occurs in the gene. Below are some key consequences:
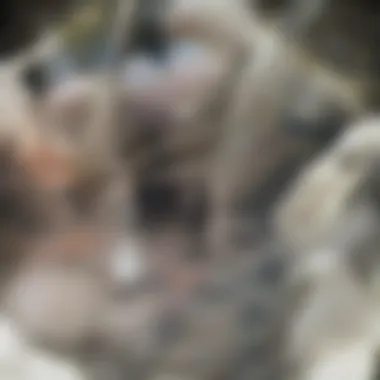
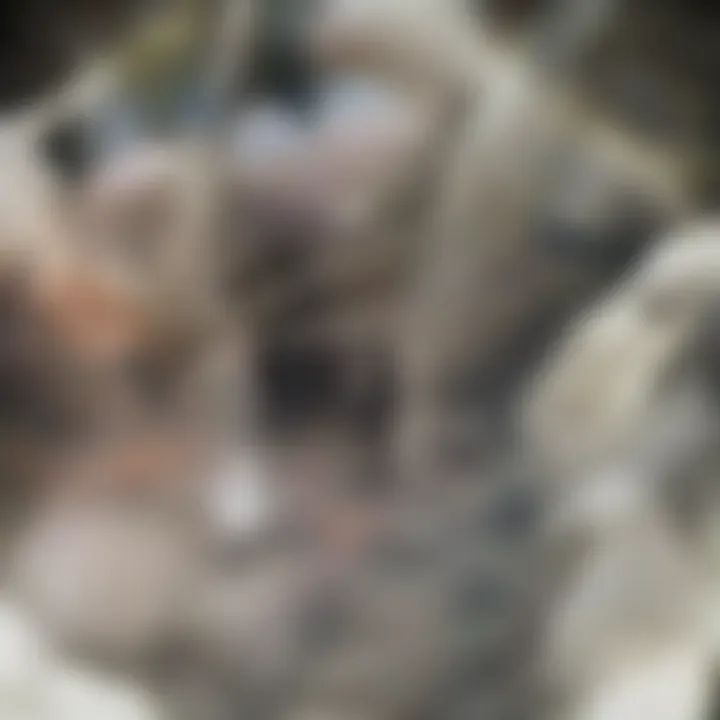
- Altered Protein Function: Missense mutations can result in proteins that have different activities, possibly impacting metabolic pathways or cellular signaling.
- Loss of Function: Nonsense mutations or frameshifts can lead to a complete loss of protein function. This can disrupt essential cellular processes that rely on that specific protein.
- Potential for Disease: Many diseases are linked to mutations. For example, sickle cell anemia is caused by a single-point mutation in the hemoglobin gene, reflecting the direct result of a missense mutation.
- Increased Protein Production: In some cases, duplication mutations lead to an increase in protein output, which can be detrimental if it causes cellular stress or disruption in regulatory pathways.
Understanding the implications of mutations is vital for grasping the broader consequences they bear on health, development, and adaptation across species.
Applications in Biotechnology
The applications of codon translation in biotechnology are vast and impactful. Understanding the mechanisms and implications of this process is essential for advancing multiple fields, including genetic engineering, drug development, and synthetic biology. Specific examples demonstrate how the intricacies of codon translation contribute to innovative solutions that address real-world challenges.
Synthetic Biology Approaches
Synthetic biology focuses on designing and constructing new biological parts, devices, and systems. Codon translation plays a pivotal role in this area. By manipulating genetic sequences, scientists can create organisms that synthesize desired proteins more efficiently.
One prominent example of this is in the development of yeast and bacteria that produce pharmaceuticals. These organisms can be engineered to express therapeutic proteins by optimizing their codons for the host organism being used.
In synthetic biology, researchers often employ techniques such as CRISPR-Cas9 for gene editing. This allows precise modifications in DNA, directly affecting the codon sequences. The improved translation efficiency often leads to better yields of the target proteins. Key considerations in these approaches include:
- Codon usage bias: Different organisms preferentially utilize specific codons. Matching the codon choices in synthetic genes to the host reduces translation errors.
- Gene assembly techniques: New methods, such as Gibson assembly, enable swift combination of multiple DNA fragments, optimizing translation across sectors.
Codon Optimization
Codon optimization is a strategic approach that enhances the efficiency of gene expression in biotechnology. By adjusting the sequence of codons in a gene to reflect the preferences of the host organism's translational machinery, scientists can significantly improve yield and activity of proteins.
Efforts in codon optimization involve several strategies:
- Codon Usage Tables: Using these tables, researchers can identify which codons are favored within a given organism and re-design sequences accordingly.
- G+C Content: Modifying the Guanine and Cytosine content can affect RNA stability and translation efficiency, enhancing overall protein yields.
- Secondary Structure of mRNA: Avoiding the formation of strong secondary structures in the mRNA can improve accessibility to the ribosomal machinery, allowing for more effective translation.
- Use of Artificial Intelligence: Novel computational methods help predict optimal codons and design sequences for maximum expression.
It is important to note the trade-offs involved in codon optimization. While improved translation may enhance protein production, it can also introduce unanticipated effects on protein folding and functionality.
In summary, the applications of codon translation in biotechnology are wide-ranging. By understanding and optimizing this process, researchers can drive advancements in multiple fields while addressing significant global challenges.
Challenges in Codon Translation Research
The study of codon translation is intricate, revealing a maze of biological processes. Understanding these challenges is crucial for advancing our comprehension of molecular biology. The translation process can be influenced by several factors that deserve attention. Research on these aspects can provide insights into the effectiveness and efficiency of protein synthesis.
Complexity of the Translation Process
Translation is a multi-step process. Each stage requires precise coordination of various molecules. Ribosomes, transfer RNA (tRNA), and messenger RNA (mRNA) all play pivotal roles. The interactions among these components can be complex to study. For instance, variations in the availability of amino acids can affect the speed and accuracy of translation. Furthermore, conditions within the cellular environment can introduce additional variables.
To understand the challenges better, consider the following factors:
- Variability in Codon Usage: Different organisms exhibit preferences for certain codons. This affects how efficiently ribosomes can translate mRNA into proteins.
- Secondary Structure of mRNA: The folding of mRNA can influence the accessibility of ribosomes to the codons, impacting the overall translation rate.
- Post-Transcriptional Modifications: Modifications to mRNA can alter its function. This can present obstacles when studying translation since modified mRNAs may behave unexpectedly.
Studying these complexities requires advanced methodologies and frameworks, which can sometimes produce inconsistent results.
Technological Limitations
Despite advancements in biotechnology, researchers face technological barriers in studying codon translation. Techniques such as high-throughput sequencing have transformed our understanding, but they also come with challenges. Data generated can be vast yet complicated. Analyzing this data demands substantial computational power and refined algorithms. Not all research labs have access to these technologies, which can limit collaborative studies.
Additionally, traditional methods may not capture the dynamic nature of translation in real-time. For example, using fluorescence microscopy can help visualize translation; however, this approach has its limits in resolving specific interactions. Some important interactions may occur too quickly or in environments that are difficult to replicate in vitro.
"Future innovations must address these limitations to explore the nuances of codon translation effectively."
This calls for interdisciplinary approaches, combining molecular biology with computational models to predict translation dynamics. This can enhance our understanding of codon translation and its implications in health and disease.
Future Directions in Research
Research in codon translation holds significant implications for advancements in molecular biology. As we navigate the complexities of protein synthesis, understanding the future directions in this field is crucial not only for basic biological understanding but also for practical applications in biotechnology, medicine, and synthetic biology.
Emerging technologies will likely play a pivotal role in these future directions. Innovations such as CRISPR-Cas9 gene editing and advancements in next-generation sequencing offer new tools for examining translation processes more closely. These technologies enable researchers to manipulate genetic sequences with unprecedented precision, revealing insights into how codon translation impacts cellular functions. By utilizing these methods, scientists are uncovering the relationship between codon usage and disease phenotypes, thus paving the way for targeted therapeutic strategies.
Additionally, studying the interactions between different biomolecules during the translation process poses another avenue for future research. Understanding these interactions can help elucidate the mechanisms of translation fidelity, and how errors in translation may lead to diseases, including various types of cancer.
Emerging Technologies
Emerging technologies present a transformative potential in the study of codon translation. Current methodologies are enhancing our ability to visualize and manipulate molecules involved in the translation process. High-resolution imaging techniques, including cryo-electron microscopy, allow scientists to observe ribosomal assemblies and interactions in real-time.
Another key development is the integration of artificial intelligence and machine learning into biological research. By leveraging AI algorithms, researchers can analyze vast datasets to identify patterns in codon usage that may correlate with specific diseases. This approach not only accelerates the discovery process but also augments our understanding of protein folding and stability, which are critical for functionality.
Benefits of these technologies include:
- Enhanced precision in studying molecular interactions
- Greater understanding of translational dynamics
- Possibility of designing novel therapeutic interventions
Interdisciplinary Approaches
The future of codon translation research also lies in interdisciplinary collaboration. By combining insights from molecular biology, bioinformatics, and systems biology, researchers can approach codon translation from multiple angles. Such collaboration may enhance our understanding of how codon sequences influence protein synthesis and function.
This collaborative environment fosters innovation by bridging gaps in knowledge. For instance, integrating computational models with experimental data can uncover the intricacies of the translation machinery and its regulation. It allows for the simulation of translation processes, predicting outcomes based on different genetic contexts.
"Interdisciplinary approaches enrich the study of codon translation, providing a holistic view that is essential for breakthroughs in molecular biology."
As researchers continue to unravel the complexities of codon translation, the potential for transformative discoveries increases. Uniting expertise from diverse disciplines will undoubtedly lead to innovative solutions for challenges in biology and medicine.