Comprehensive Guide to PCR Steps in Molecular Biology
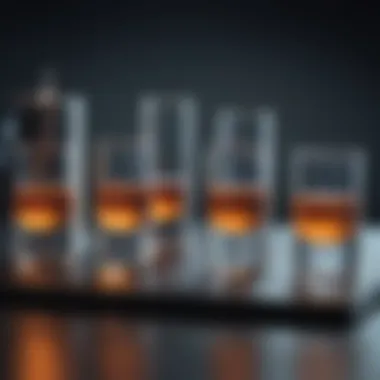
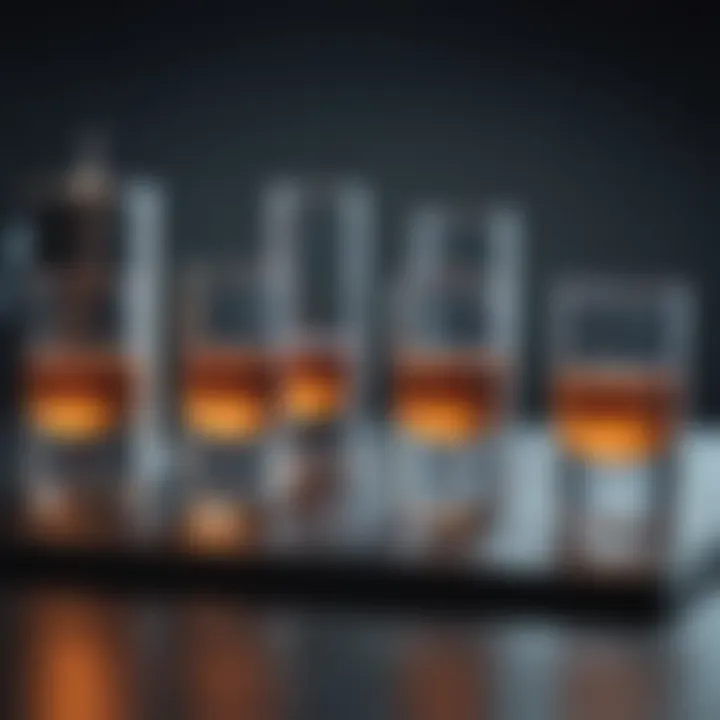
Research Overview
The Polymerase Chain Reaction (PCR) is a fundamental technique in molecular biology, crucial for amplifying DNA sequences. This section aims to provide an insightful summary of the research and its methodologies.
Key Findings
One of the most significant findings in the field of PCR encompasses its ability to amplify minute quantities of DNA. The versatility of PCR has led to its adoption in various applications, ranging from genetic research to clinical diagnostics. Specific findings, including the sensitivity and specificity of PCR protocols, reveal their efficacy in detecting genetic mutations and infectious agents. Researchers have noted that the advancement in thermal cycling technology has greatly improved the reliability of PCR results.
Study Methodology
The method of PCR involves three primary steps: denaturation, annealing, and extension. In numerous studies, researchers have utilized a variety of DNA templates, primers, and polymerases to optimize these parameters. Key controlled factors include temperature, time, and reagent concentrations. The systematic exploration of these variables enables researchers to develop more robust and efficient PCR processes.
Background and Context
To appreciate the utility of PCR, one must consider its historical context and contemporary relevance.
Historical Background
PCR was invented by Kary Mullis in 1983, a breakthrough that transformed genetics and molecular biology. This technique emerged as a response to the need for rapid and efficient methods to amplify DNA. Mullis's original method has undergone several refinements, adapting it for advanced molecular techniques, including quantitative PCR and reverse transcription PCR.
Current Trends in the Field
Today, PCR continues to evolve. Current trends involve the integration of PCR with next-generation sequencing technologies. This synergy allows for comprehensive genomic analysis and high-throughput screening. Another trend is the development of digital PCR, which offers unparalleled precision in measuring DNA quantities. Furthermore, the rise of multiplex PCR techniques enables the simultaneous amplification of multiple targets within a single reaction, broadening the scope of possible applications.
"PCR's impact on science and medicine cannot be overstated. It is a powerful tool that continues to innovate DNA analysis, diagnostics, and research fundamentals."
By understanding these components, researchers and practitioners alike can leverage PCR more effectively in their studies, ultimately driving forward the boundaries of molecular biology.
Overview of PCR
Polymerase Chain Reaction (PCR) is a cornerstone technique in molecular biology that serves multiple critical functions, from amplifying specific DNA sequences to facilitating numerous applications in diverse fields. Understanding PCR is paramount for students, researchers, and professionals who navigate biological sciences or related disciplines.
PCR allows scientists to generate millions of copies of a particular DNA segment quickly and efficiently. This capability transforms how research is conducted, as it provides sufficient material for analysis, enabling detailed study of the genetic code, disease mechanisms, and more.
History and Development
The history of PCR is relatively brief yet transformative. The technique was invented in 1983 by Kary Mullis, who envisioned a method that could exponentially amplify DNA. Before the advent of PCR, DNA analysis was a laborious task, often requiring substantial amounts of starting material. Mullis' innovation simplified this process through thermal cycling, which later became the foundation for many laboratory protocols.
PCR rapidly gained traction in laboratory settings and evolved significantly over the subsequent decades. Various modifications have been introduced to improve efficiency and specificity. For instance, the development of Taq polymerase, an enzyme derived from the heat-resistant bacterium Thermus aquaticus, allowed for more stable and effective DNA amplification during thermal cycling. Today, PCR is not only standard in research and diagnostics but also serves as a foundational technology in fields such as biotechnology and forensic science.
Importance of PCR in Modern Science
The significance of PCR in modern science cannot be overstated. It is a versatile tool that has applications in clinical diagnostics, genetic research, forensic analysis, and even environmental studies. PCR enables rapid diagnostics for infectious diseases, supports genetic engineering efforts, and aids in understanding genetic disorders.
PCR's impact extends beyond the laboratory. It plays a pivotal role in public health, agriculture, and conservation efforts. With the integration of advanced techniques like real-time PCR and multiplex PCR, scientists can analyze multiple targets simultaneously, enhancing the depth of genetic studies.
"PCR has reshaped the landscape of molecular diagnostics and research, enabling breakthroughs in understanding biology and disease."
In summary, the overview of PCR encapsulates its history, significance, and fundamental role in scientific advancements. The subsequent sections will delve deeper into the intricate steps of PCR, the foundational concepts, and the detailed phases of the process.
With each step, we will unravel how PCR continues to influence scientific research and applications across various fields.
Fundamental Concepts of PCR
The understanding of fundamental concepts in Polymerase Chain Reaction (PCR) is essential for anyone working in molecular biology. It lays the groundwork for grasping the significance of each part of the PCR process. This understanding helps in troubleshooting and optimizing the PCR reactions for various applications, such as clinical diagnostics, research, and forensic science.
By knowing the basic principles and components of PCR, practitioners can enhance the efficiency of DNA amplification and ensure reliable results.
Basic Principles of PCR
PCR is a technique used to amplify specific DNA sequences. Its primary principle involves repeated cycles of denaturation, annealing, and extension. During denaturation, the DNA double helix separates into two single strands. Next, primers bind to their complementary sequences during annealing. Finally, DNA polymerase synthesizes new DNA strands during the extension phase. Each cycle effectively doubles the amount of DNA, leading to exponential amplification. This process allows scientists to obtain millions of copies of a target DNA sequence, which can be utilized in various applications.
Components Required for PCR
To carry out a successful PCR, several crucial components are necessary. Understanding these components can improve reaction quality and outcomes.
DNA Template
The DNA template provides the segment of DNA that needs amplification. Its quality and purity significantly impact PCR results. A high-quality DNA template ensures that there are sufficient amounts of intact nucleic acid for the DNA polymerase to work with. Contaminated or degraded DNA can lead to unsuccessful amplification or nonspecific products. It is important to select DNA sources, such as genomic DNA, plasmid DNA, or cDNA, suitable for the intended application.
Primers
Primers are short sequences of nucleotides, typically between 18 and 25 base pairs long, designed to initiate DNA synthesis. They are key for targeting specific DNA regions. The specificity of the primers determines the precision of the PCR. Primers must be complementary to the DNA sequence flanking the target region, which allows them to anneal during the PCR process. Designing effective primers requires careful consideration of factors like melting temperature, specificity, and potential secondary structures to ensure optimal amplification.
DNA Polymerase
DNA polymerase is the enzyme responsible for synthesizing new DNA strands by adding nucleotides complementary to the DNA template. The choice of polymerase is important; for instance, Taq polymerase is commonly used because of its heat stability, allowing it to function at the high temperatures used during PCR. Other enzymes offer different properties, such as proofreading ability, which reduces errors in newly synthesized DNA. Selecting the right polymerase for specific applications can improve both the yield and fidelity of DNA amplification.
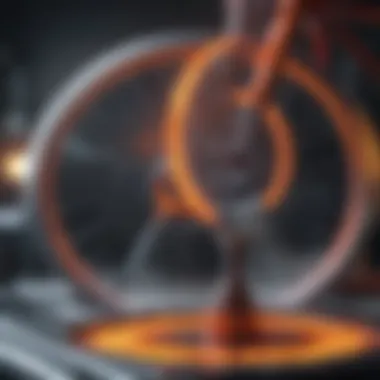
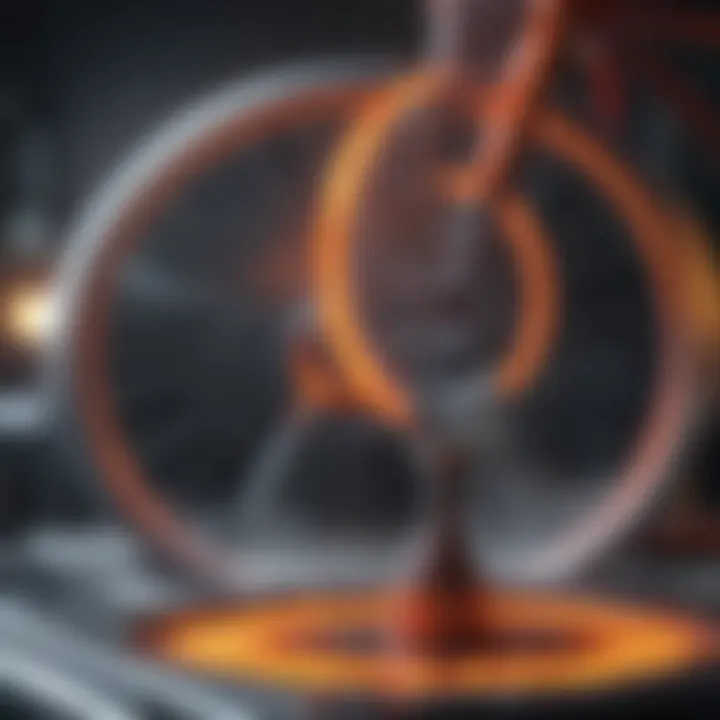
Nucleotides
Nucleotides serve as the building blocks of DNA strands. They are incorporated into the growing DNA strand by DNA polymerase. A typical PCR reaction contains four types of nucleotides: adenine (A), cytosine (C), guanine (G), and thymine (T). The concentration of nucleotides needs to be balanced, as too low a concentration can limit amplification, while too high can lead to non-specific reactions. Having an optimal supply of nucleotides is vital for achieving a successful PCR.
Buffer Solutions
Buffer solutions are critical in maintaining the optimal pH and ionic strength for the PCR reaction. A common buffer used is Tris-HCl, which helps stabilize the reaction conditions. The buffer contributes to enzyme activity and ensures that all components interact effectively. It also helps minimize the effects of contaminants and other variables that can inhibit PCR. A well-optimized buffer can improve reaction efficiency significantly.
Step-by-Step Breakdown of the PCR Process
The Polymerase Chain Reaction (PCR) is a foundational technique in molecular biology that allows for the amplification of specific DNA sequences. Each step in the PCR process is crucial to ensure accurate and effective replication of the DNA. Understanding the systematic breakdown of this process helps elucidate both practical applications and the principles driving this technique. The step-by-step approach not only highlights the sequential nature of PCR but also underscores the importance of precise temperature control, reagent balance, and timing, which ultimately contribute to the efficiency of the DNA amplification.
Preparation of the Reaction Mixture
The preparation of the reaction mixture is a critical first step in the PCR process. It involves combining the essential components needed for the reaction to take place. These components include a DNA template, primers, DNA polymerase, nucleotides, and buffer solutions. The careful selection and concentration of these elements is paramount to achieving successful amplification. A poorly prepared reaction mixture can lead to inefficiencies, such as low yield or non-specific amplification, which can negatively impact the results.
When preparing the mixture, it is vital to keep all reagents on ice and work quickly to avoid degradation or contamination. Each component plays a specific role:
- DNA Template: The sample containing the DNA sequence to be amplified.
- Primers: Short sequences of nucleotides that provide a starting point for DNA synthesis.
- DNA Polymerase: The enzyme responsible for adding nucleotides to the growing DNA strand during the extension phase.
- Nucleotides: The building blocks of DNA, which are incorporated into the new strands.
- Buffer Solutions: Maintain optimal pH and ionic conditions for the reaction to proceed.
Thermal Cycling Phases
The thermal cycling phases are the heart of the PCR process. This series of controlled temperature changes ensures the effective amplification of the DNA. The cycling typically consists of three main phases: denaturation, annealing, and extension. Each of these phases serves a distinct function essential to DNA amplification.
Denaturation Phase
The denaturation phase is usually the first step in the thermal cycling process. It typically occurs at a high temperature, around 94-98 degrees Celsius, for about 20 to 30 seconds. During this phase, the double-stranded DNA template is heated to separate it into two single strands. This strand separation is critical because it creates two distinct templates for the annealing of primers.
The key characteristic of the denaturation phase is its ability to disrupt the hydrogen bonds between nucleotide bases, enabling access to the template strands. This phase is a beneficial choice for this article as it is foundational to the PCR methodology. A key advantage is that this temperature range ensures nearly complete denaturation of most DNA templates, creating optimal conditions for the subsequent phases.
Annealing Phase
Once denaturation is complete, the temperature is lowered to allow primers to bind to their complementary sequences on the single-stranded DNA. The optimal annealing temperature usually ranges between 50 to 65 degrees Celsius, depending on the melting temperature of the primers. This phase lasts for about 20-40 seconds.
The significance of the annealing phase lies in its role in target sequence specificity. A carefully chosen annealing temperature helps ensure that primers bind specifically to the intended regions of the template DNA. This specificity is vital to reducing the likelihood of non-specific amplification, which can lead to erroneous results. The unique feature of this phase is the delicate balance between temperature and time, which can affect amplification efficiency.
Extension Phase
The extension phase follows annealing. Here, the temperature is typically set to about 72 degrees Celsius, which is the ideal temperature for the action of DNA polymerase, usually Taq polymerase. This phase lasts for 30 seconds to several minutes, depending on the length of the DNA to be amplified.
During the extension phase, DNA polymerase synthesizes new DNA strands by adding nucleotides to the 3' end of the primers. The key characteristic of this phase is the enzymatic activity of DNA polymerase. The distinct advantage of this extension temperature is that it enhances the efficiency of DNA synthesis. The ability to tailor the duration of this stage allows researchers to optimize for larger fragments or fine-tune the protocol for specific applications.
In summary, the step-by-step breakdown of the PCR process is invaluable for understanding the intricacies of this powerful technique. Each phase of PCR serves a purposeful function that collectively contributes to the accurate amplification of DNA sequences. Getting familiar with these phases and their importance within the overall PCR methodology allows for troubleshooting and refinement of protocols applied in various scientific fields.
Denaturation Phase Explained
The denaturation phase is a crucial step in the Polymerase Chain Reaction (PCR) process. It marks the initiation of DNA amplification. During this phase, the double-stranded DNA undergoes a transformation that is essential for subsequent steps of PCR. Without effective denaturation, the ability to target and amplify specific DNA sequences is severely compromised.
Temperature Conditions
Optimal temperature conditions are vital for effective denaturation. Generally, the temperature is raised to about 94 to 98 degrees Celsius. This high temperature is necessary to disrupt the hydrogen bonds that hold the two strands of DNA together. The result is the separation of the double helix into two single strands.
Maintaining the correct temperature ensures that the DNA template is adequately denatured without damaging the integrity of the molecule. If the temperature is too low, the strands may not fully separate. Conversely, excessive heating can lead to degradation of the DNA and loss of template material. Close attention to temperature control is therefore paramount.
Mechanism of Strand Separation
The mechanism of strand separation during the denaturation phase relies on the principles of heat-induced disruption of molecular interactions. As the temperature rises, the kinetic energy within the DNA molecules increases. This energy overcomes the forces binding the two strands together. Each DNA base pair connects via hydrogen bonds, and under the denaturation conditions, these bonds break apart.
This separation facilitates the access of primers to their specific target sequences during the next phase of PCR. It is important to note that proper denaturation enables efficient amplification of the DNA. Without successful strand separation, the entire PCR process would fail.
Effective denaturation is essential for the success of the PCR process, as it sets the stage for primer annealing and DNA synthesis.
In summary, the denaturation phase plays a significant role in PCR by ensuring that DNA strands are properly separated. This allows for optimal conditions for the subsequent annealing and extension phases, leading to successful amplification of the desired DNA sequences. Understanding this phase is essential for anyone involved in molecular biology, as it lays the foundation for all subsequent steps.
Annealing Phase Explained
The annealing phase is a critical step in the Polymerase Chain Reaction (PCR) process. It follows the denaturation phase, where the double-stranded DNA has been separated into two single strands. During annealing, the temperature of the reaction mixture is reduced, allowing the primers to bind to their respective complementary sequences on the single-stranded DNA template. This specific pairing is crucial, as it sets the stage for the next step in the PCR process: the extension phase.
Selection of Optimal Temperature
Selecting the optimal temperature for the annealing phase significantly influences the success of the PCR reaction. Typically, this temperature ranges from 50°C to 65°C, depending on the melting temperature (Tm) of the primers used. The Tm is determined by the length and composition of the primers. If the temperature is too high, the primers may not bind effectively to the target sequence, resulting in poor amplification. Conversely, if the temperature is too low, non-specific binding can occur. This can lead to the amplification of unintended sequences, which complicates downstream applications.
To find the optimal annealing temperature, researchers often perform a gradient PCR. This technique tests several temperatures within the suggested range to determine which results in the most specific amplification of the target DNA sequence. Having precise conditions facilitates the reproducibility of experiments and improves the overall efficiency of the PCR.
Role of Primers in Targeting Sequences
Primers play an essential role in defining the specificity of the PCR process. These are short, single-stranded sequences of nucleotides, designed to complement the target region of the DNA template. During the annealing phase, the efficiency and accuracy of the primers' binding are critical because they dictate which segments of DNA will be amplified.
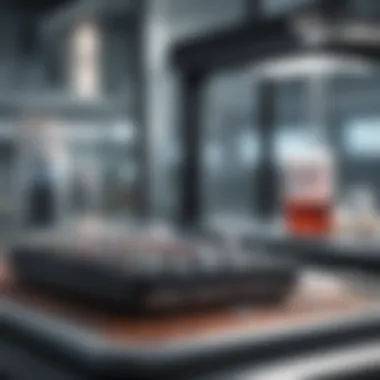
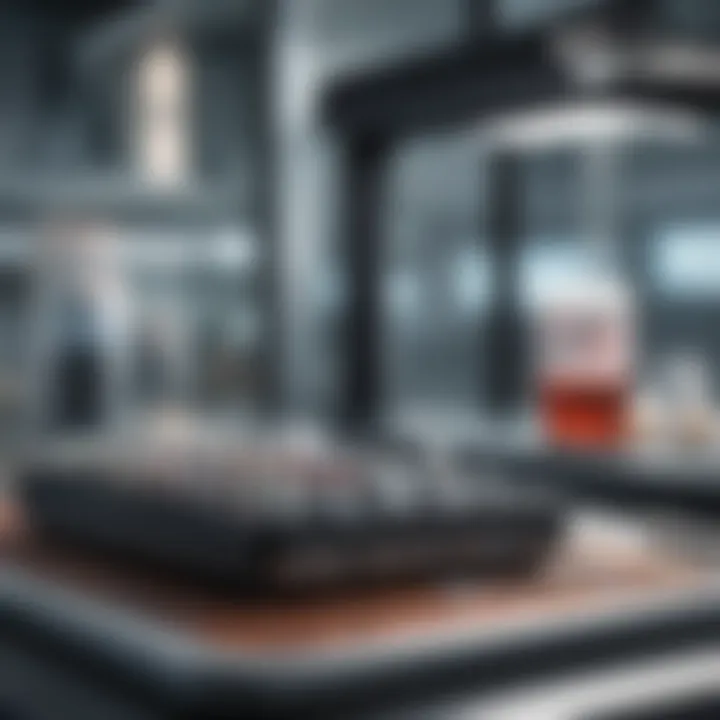
The choice of primers affects several aspects of PCR:
- Specificity: Well-designed primers bind only to the intended target sequence, preventing amplification of non-target regions.
- Efficiency: Primers should be designed for optimal length and concentration to reduce the chances of primer-dimer formation, which can inhibit the amplification process.
- Sensitivity: A higher affinity of primers for the target sequence leads to better amplification, making the PCR more sensitive to detect low copy numbers.
In summary, during the annealing phase, both the selection of optimal temperature and the role of primers are fundamental for the overall success of PCR. Proper attention to these factors enhances the precision and reliability of PCR applications across various fields, from clinical diagnostics to forensic science.
Extension Phase Explained
The extension phase is a critical step in the Polymerase Chain Reaction (PCR) process. This phase is significant for generating new DNA strands from the template provided. It occurs after the denaturation and annealing phases, during which the DNA polymerase enzyme actively synthesizes complementary DNA strands. This process is essential for amplifying the target DNA sequences, therefore enabling numerous downstream applications in molecular biology, forensic science, and medical diagnostics.
Function of DNA Polymerase
DNA polymerase serves as the fundamental enzyme in the extension phase. Its primary role is to catalyze the addition of nucleotides to the growing DNA strand. Different types of DNA polymerases can be utilized in PCR, with Taq polymerase being the most popular due to its ability to withstand high temperatures during the denaturation phase. Taq polymerase can elongate the DNA strand at a typical rate of about 1000 nucleotides per minute.
This phase occurs at optimal temperatures, generally around 72 degrees Celsius for Taq polymerase. The precise temperature will depend on the specific enzyme being used. If the temperature is too low, the enzyme may not function efficiently. Conversely, excessively high temperatures could lead to a denaturation of the enzyme itself, disrupting the PCR process.
Effective execution of the extension phase ensures that ample amounts of target DNA are synthesized for analysis and further experimentation.
Synthesis of Complementary Strands
During the synthesis of complementary strands, DNA polymerase moves along the template strand, adding nucleotides to the growing strand. Nucleotides are incorporated based on the complementary base pairing rules—adenine pairs with thymine, and cytosine pairs with guanine. This step is instrumental in creating a double-stranded DNA product from a single-stranded template.
This phase varies in duration based on the length of the target sequence being amplified. Typically, it lasts from 30 seconds to several minutes. In cases where longer sequences need to be amplified, longer extension times may be required, but this could extend overall PCR cycling times.
Factors Affecting PCR Efficiency
Understanding the factors affecting PCR efficiency is crucial for optimizing this powerful technique. It directly influences the accuracy of the results, protocol reproducibility, and overall efficacy in DNA amplification. Researchers and practitioners must be aware of several key factors that can enhance or inhibit the PCR process. Key elements include the quality of the DNA template, concentration of reagents, and thermal cycle parameters. Addressing these considerations can lead to more reliable outcomes in both research and clinical applications.
Quality of DNA Template
The quality of the DNA template is perhaps the most significant factor influencing PCR efficiency. If the template DNA is degraded or contaminated, the amplification can significantly suffer. High-quality DNA is characterized by purity, which means it should be free from inhibitors, proteins, and other contaminants.
To evaluate the quality, one can inspect the DNA using spectrophotometric methods or gel electrophoresis. A variety of factors, such as the source of the DNA and the isolation methodology, dictate the end quality of the DNA. For example, DNA extracted from formalin-fixed, paraffin-embedded tissues may present more challenges compared to fresh tissue samples. Here are crucial points to consider regarding the DNA template:
- Integrity: Intact double-stranded DNA provides a better basis for amplification.
- Concentration: The appropriate concentration enhances amplification without leading to non-specific results.
- Purity: AOD ratios (260/280 nm and 260/230 nm) indicate the presence of contaminants that may inhibit PCR.
Concentration of Reagents
Next, the concentration of reagents has a direct effect on PCR results. This includes the amount of primers, nucleotides, and polymerase used in the reaction mix. If the concentrations are too low, the reaction may not amplify the target sequence effectively. Conversely, excessive concentrations can result in non-specific amplification due to primer-dimer formation.
For priming:
- Primers - Typically, a concentration between 0.1 to 0.5 µM is recommended. The specific concentration may vary based on the complexity and the size of the target sequence.
- Nucleotides - A standard final concentration of 200 µM for each nucleotide is common to sustain the reaction.
- DNA Polymerase - The enzyme's concentration directly impacts the performance of the amplification. Too little may decrease yield, while too much may enhance errors in the extension phase.
Thermal Cycle Parameters
Finally, the thermal cycling parameters play a crucial role in the success of PCR reactions. This encompasses the temperature settings and the duration allocated for each phase of the PCR process. Each step must be optimized to facilitate the best yield.
- Denaturation Temperature: Typically set between 94 to 98 degrees Celsius; too low can result in incomplete strand separation.
- Annealing Temperature: Careful calibration here is necessary. It should be lower than the melting temperature of the primers to ensure proper binding, usually between 50 to 65 degrees Celsius.
- Extension Time and Temperature: Depending on the DNA polymerase used, the extension time may vary. A general rule is 1 minute for every 1 kb of DNA at 72 degrees Celsius.
Optimizing these parameters leads to improved specificity and yield in PCR amplification. An understanding of how each of these factors interact allows for better planning of experimental procedures, making PCR a more reliable tool for molecular analysis.
Thus, ensuring the right balance of template quality, reagent concentration, and thermal cycling settings can significantly improve PCR efficiency.
Applications of PCR
Polymerase Chain Reaction (PCR) has become a cornerstone in molecular biology, providing tools that unlock vast fields of research as well as practical applications in various areas. The significance of PCR extends far beyond mere amplification of DNA; it enables rapid and effective analysis that can influence clinical practices, forensic investigations, and innovative scientific research. Understanding the applications of PCR reveals its transformative impact on many disciplines.
Clinical Diagnostics
PCR plays a crucial role in clinical diagnostics, particularly in the identification of pathogens. Infections caused by viruses, bacteria, and other microorganisms can be detected with high sensitivity and specificity using PCR techniques. By amplifying specific sequences of the pathogen’s DNA, PCR allows for the early diagnosis of diseases such as HIV, tuberculosis, and many others. The rapid turnaround time associated with PCR is vital in clinical settings, enabling timely treatment decisions.
Benefits of PCR in clinical diagnostics include:
- High sensitivity: Ability to detect low levels of target DNA.
- Speed: Quickly delivers results that are essential for patient care.
- Specificity: Reduces the likelihood of false positives.
Through techniques such as reverse transcription PCR (RT-PCR), healthcare professionals can not only detect viral RNA but also gauge viral load, which is critical in monitoring infections over time. This has significant implications for both patient management and population health surveillance.
Forensic Science
In the realm of forensic science, PCR is invaluable for DNA profiling. It allows forensic experts to analyze minute amounts of biological material, often recovered from crime scenes. A single hair, drop of blood, or any biological sample can be amplified sufficiently for accurate analysis.
Key aspects of PCR in forensics include:
- Identification of suspects: Matching DNA profiles from crime scene evidence to subjects.
- Exclusion of individuals: Quickly ruling out suspects based on DNA testing.
- Cold cases: Re-analyzing old evidence with modern PCR methods offers a chance at solving past crimes.
PCR has revolutionized the field of forensic science, enhancing the accuracy and reliability of investigations. With its ability to amplify degraded or scant DNA, PCR opens new avenues in cold case investigations and inBuilding public trust in forensic evidence.
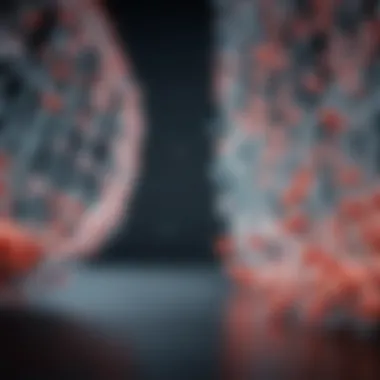
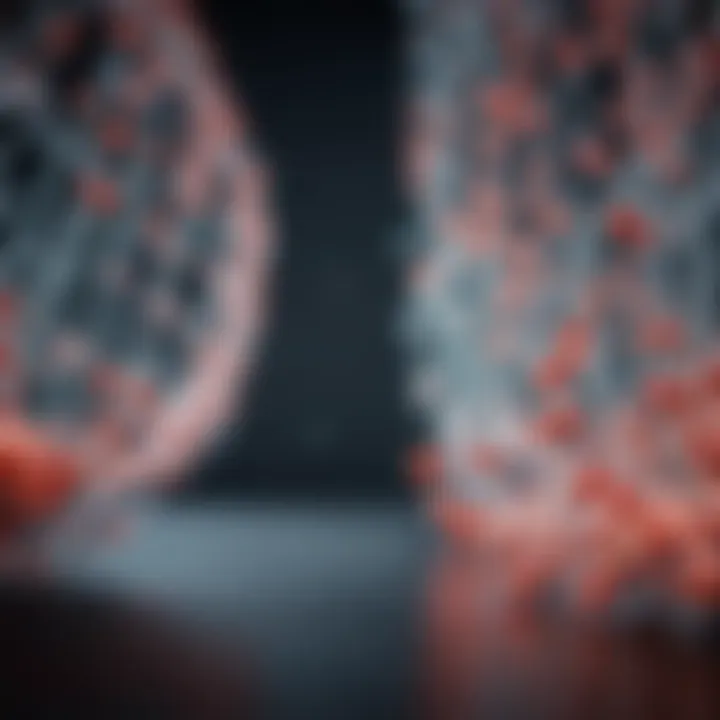
Research and Development
Beyond diagnostics and forensics, PCR is pivotal in research and development. It is a fundamental tool used in various research fields, such as genetics, microbiology, and biotechnology. Through gene cloning, sequencing, and mutagenesis, researchers utilize PCR to manipulate and study genetic material in a controlled manner.
Important applications of PCR in research include:
- Gene expression studies: Investigating the regulation of genes in different conditions.
- Genetic engineering: Creating genetically modified organisms (GMOs) for agricultural improvements.
- Pathogen detection: Monitoring the presence of pathogens in environmental samples.
PCR's ability to provide quantitative data in real-time PCR assays has further enhanced its application in research. By allowing researchers to monitor DNA amplification during the PCR process, scientists can assess gene expression levels with precision and reliability.
"PCR technology has reshaped our understanding of molecular biology, facilitating advancements in diagnostics, forensics, and research that were previously unimaginable."
Challenges and Limitations of PCR
While Polymerase Chain Reaction (PCR) is a powerful tool in molecular biology, it is not without challenges and limitations that can affect the overall effectiveness and accuracy of results. Understanding these issues is crucial for anyone undertaking PCR, as they can significantly influence both experimental outcomes and interpretations.
Contamination Issues
Contamination is one of the most significant challenges in PCR. The sensitivity of the technique means that even minute amounts of extraneous DNA can lead to false positives. This contamination can arise from various sources:
- Environmental Contaminants: PCR reaction mixtures can easily pick up DNA from the surrounding environment, including dust, skin cells, and even airborne particulates.
- Reagent Contamination: The ingredients used in PCR, like primers and enzymes, can sometimes harbor trace levels of contaminants if not properly handled or stored.
- Cross-Contamination: In laboratory settings, samples can inadvertently mix due to mishandling or inadequate cleaning procedures.
To mitigate contamination risks, several practices can be implemented: use of sterile equipment, working in dedicated PCR areas, and regular cleaning of work surfaces. Additionally, implementing positive and negative controls in each experiment helps to identify contamination early in the process, ensuring the validity of the results.
Specificity and Sensitivity Concerns
PCR's success hinges on its specificity and sensitivity, yet acquiring both can be challenging. Specificity refers to the ability of PCR to amplify only the target DNA sequence, while sensitivity pertains to the detection of low concentrations of DNA.
- Primer Design: Poorly designed primers can bind to non-target sequences, resulting in non-specific amplification. Selecting high-quality primers that match the target DNA closely enhances specificity.
- Reaction Conditions: Optimizing temperature and salt concentrations is vital for maintaining specificity. If annealing temperature is too low, primers may bind to incorrect sites and results will be misleading.
- Template Quality: The integrity of the DNA template is paramount; degraded DNA can produce erratic amplification results and lead to incorrect conclusions.
A careful balance between specificity and sensitivity is essential in PCR. Researchers often need to customize their PCR conditions to the nature of their specific experiment. By addressing these concerns systematically, they can improve both the reliability and accuracy of PCR outcomes.
"The power of PCR lies not just in its ability to amplify, but in the precision of its implementation."
Innovations in PCR Technology
Innovations in PCR technology have played a crucial role in advancing molecular biology. This area of research continuously evolves, leading to significant enhancements in efficiency, specificity, and application versatility. The newest techniques bring unique advantages to both research and diagnostic implications. Understanding these innovations is essential for those engaged in molecular studies, enabling them to leverage state-of-the-art methodologies effectively.
Real-Time PCR Advances
Real-time PCR, or quantitative PCR (qPCR), represents a substantial advancement in PCR technology. It allows for the detection and quantification of DNA in real time. This functionality is achieved through the use of fluorescent dyes or probes, which emit signals as the DNA amplifies during the cycles. The key benefit of this approach is the ability to monitor the amplification process without needing to open the reaction tubes, reducing contamination risks significantly.
Key advantages of real-time PCR include:
- Dynamic quantification: This technique enables precise quantification of nucleic acids, which is essential for applications in gene expression analysis and viral load measurement.
- Speed: Real-time PCR often shortens the time required for results compared to traditional methods since it integrates amplification and detection in one step.
- High sensitivity and specificity: Real-time PCR provides a robust mechanism for detecting low-abundance targets, making it useful in applications such as pathogen detection.
As the technology advances, developments in software and instruments will continue to improve the accuracy and reliability of real-time PCR. This ensures it remains a preferred method in both research and clinical settings.
Multiplex PCR Techniques
Multiplex PCR is another key innovation that allows multiple targets to be amplified in a single reaction. This method employs more than one set of primers, enabling the detection of various sequences simultaneously. This capability significantly increases the throughput of PCR experiments and is particularly useful in diverse applications, ranging from diagnostics to genetic studies.
The benefits of multiplex PCR include:
- Efficiency: Multiple targets can be detected within one test, saving time and reagents.
- Enhanced diagnostic capabilities: It is particularly advantageous in clinical settings where detecting several pathogens or genetic variations at once is necessary.
- Reduced risk of contamination: Performing fewer reactions decreases the likelihood of cross-contamination, which is critical in sensitive experiments.
However, designing multiplex PCR requires careful consideration of primer specificity and efficiency to avoid non-specific amplification. The development of innovative software tools and optimized reagents continues to improve the viability and application of multiplex PCR in various fields.
"Innovations in PCR technology not only enhance the reliability of results but also broaden the scope of applications in research and diagnostics."
Future Directions in PCR Research
The evolution of Polymerase Chain Reaction (PCR) is not merely constrained to its foundational principles and protocols. The realm of future directions in PCR research holds immense potential for enhancing its utility in various fields, particularly in diagnostics and biological research. As technology advances, the demand for more efficient, cost-effective, and accurate PCR methods has grown. This section will delve into two key areas shaping the future of PCR research: expanding applications and integration with other biotechnologies.
Expanding Applications
PCR has become integral to numerous sectors, including clinical diagnostics, forensic science, and genetic research. However, future applications can push these boundaries even further.
- Personalized Medicine: One promising area is personalized medicine. PCR can be tailored to identify specific genetic markers in patients. This assistance in creating customized treatment plans could greatly improve health outcomes.
- Environmental Monitoring: Another intriguing application is in environmental science, where PCR can be employed to detect pathogens in water or soil samples. Identifying these microbial communities accurately can contribute to public health initiatives.
- Agricultural Biotechnology: PCR could also play a pivotal role in agriculture. By allowing for the genetic modification of crops, it enables the development of disease-resistant plants or those with enhanced nutritional profiles.
Advancements in PCR techniques, such as Loop-mediated Isothermal Amplification (LAMP), create possibilities for rapid and on-site testing. This versatility enhances PCR’s effectiveness across diverse fields and may lead to innovative solutions for global challenges.
Integration with Other Biotechnologies
The integration of PCR with other biotechnological tools is set to amplify its capabilities. Such collaborations can enhance sensitivity, specificity, and speed.
- Next-Generation Sequencing (NGS): Combining PCR with next-generation sequencing can allow researchers to quickly analyze large amounts of genetic data. This synergy can expedite the identification of variants associated with diseases.
- CRISPR Technology: The intersection of PCR and CRISPR gene editing represents a powerful combination. PCR can be utilized to confirm successful edits at specific loci, streamlining the gene editing process.
- Digital PCR Technologies: Digital PCR offers a more precise quantification of nucleic acids. Integrating this with standard PCR protocols can increase the accuracy of results, making it crucial for applications in clinical diagnostics.
In summary, the future directions in PCR research are varied and promising. Expanding applications in fields like personalized medicine and environmental monitoring will broaden the impact of PCR significantly. Meanwhile, collaboration with other biotechnologies will forge new avenues for accuracy and efficiency. Maintaining a focus on these developments is essential for maximizing the potential of PCR as a critical tool in the scientific community.
"The future of PCR is not just in its continued use, but in its evolution and integration with other leading-edge technologies. The paradigm of research is shifting, and so must our techniques."
For more information on PCR advancements, visit Wikipedia and Britannica.