Exploring CRISPR-Cas9 Knock-In Cell Lines
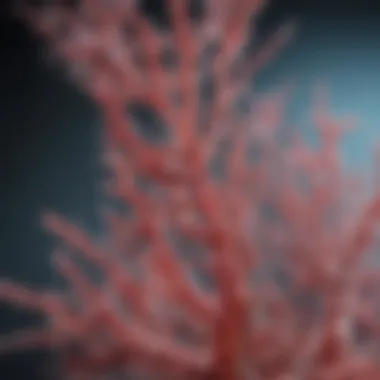
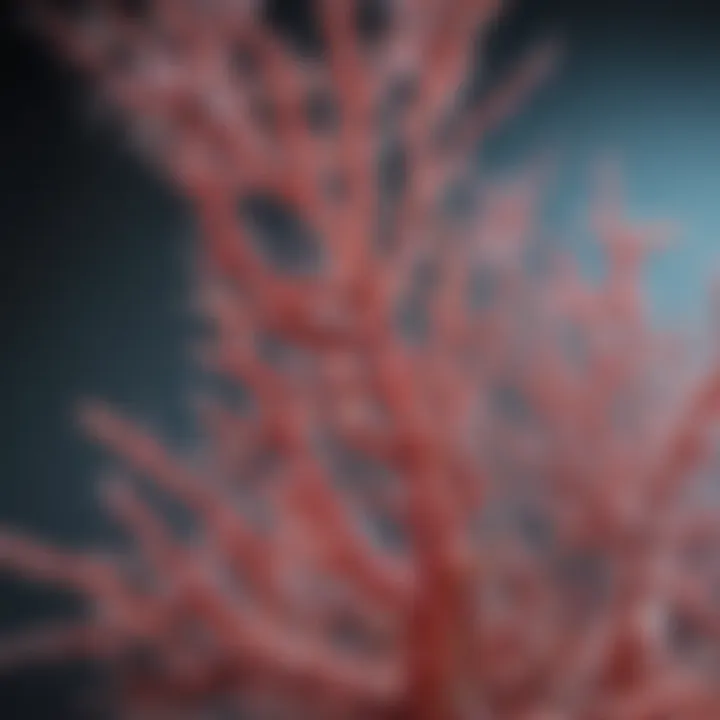
Research Overview
CRISPR-Cas9 has revolutionized the field of genetic engineering, particularly through its application in creating knock-in cell lines. This technology allows for precise modifications at specific locations in the genome, enabling researchers to insert genes of interest into existing genetic sequences. Such advancements offer new horizons in understanding gene function, exploring disease mechanisms, and developing therapeutic strategies.
Key Findings
Recent studies highlight the following key aspects of CRISPR-Cas9 knock-in technology:
- Precision and Efficiency: CRISPR-Cas9 knock-in techniques are highly precise compared to traditional methods, such as homologous recombination. This precision minimizes off-target effects and improves the reliability of results.
- Versatile Applications: The ability to create specific genetic modifications opens up a range of applications from drug development to studying genetic diseases. Knock-in cell lines can serve as valuable models for cancer research, hereditary disorders, and more.
- Ethical Considerations: With immense power comes great responsibility. Ethical debates surrounding gene editing raise questions about the implications of modifying living organisms, especially concerning potential long-term effects on ecosystems and human health.
Study Methodology
The methodologies employed in CRISPR-Cas9 experiments are intricate and involve several critical steps:
- Guide RNA Design: The first step involves designing guide RNAs that are complementary to the target gene sequence, assuring specificity of the Cas9 enzyme.
- Cell Line Selection: Appropriate cell lines are chosen based on the research goals. This could range from human embryonic stem cells to specific cancer cell lines.
- Transfection Process: The introduction of the Cas9 enzyme and guide RNAs into the target cells frequently utilizes electroporation or lipofection methods to enhance uptake efficiency.
- Selection and Screening: After the editing process, cells that have successfully integrated the knock-in sequence must be selected. This often involves antibiotic selection and subsequent validation using PCR and sequencing.
Background and Context
Historical Background
The CRISPR-Cas9 system was discovered in bacteria, originally playing a defensive role against viruses. In recent years, scientists adapted this natural mechanism for genome editing, which has since become a cornerstone in genetic research. The publication of the groundbreaking paper by Jennifer Doudna and Emmanuelle Charpentier in 2012 was a definitive moment, setting off a flurry of applications across multiple fields.
Current Trends in the Field
Today, the landscape of CRISPR technology is rapidly evolving. Key trends include:
- Expanded Target Range: Advances have led to the development of modified Cas proteins with broader target capabilities, allowing for edits in previously difficult-to-access regions of the genome.
- Base Editing and Prime Editing: New techniques like base editing further refine gene editing by allowing single nucleotide changes without double-strand breaks, reducing potential complications.
- Emphasis on Ethical Frameworks: There’s an increasing focus on creating ethical guidelines and regulatory frameworks to oversee the use of CRISPR technology in human subjects and the environment.
"The power of CRISPR-Cas9 has sparked discussions that straddle science and ethics, invoking philosophical questions we must now confront."
As this revolutionary tool continues to shape genetic research, its implications extend far beyond the laboratory. By thoroughly studying and understanding the technologies and methodologies involved in CRISPR-Cas9 knock-in cell lines, researchers can not only advance biotechnology but also navigate the ethical dimensions of their innovations.
Preamble to CRISPR-Cas9
The field of genetics has undergone seismic shifts in recent years, and at the heart of this revolutionary change is CRISPR-Cas9 technology. This narrative will explore the pivotal role CRISPR-Cas9 plays in modern gene editing, particularly in the context of knocking in specific genetic sequences to create tailored cell lines. Understanding this topic is crucial for students, researchers, educators, and professionals, as it offers insights into the mechanics of genetic manipulation, the potential applications of such techniques, and the ethical considerations tied to them.
The significance of CRISPR-Cas9 can hardly be overstated. It provides a more accurate, efficient, and cost-effective means of editing genomes compared to previous technologies. This article will delve into the intricacies of CRISPR-Cas9, exploring how it allows for targeted modifications that were once deemed nearly impossible.
Overview of CRISPR Technology
CRISPR, short for Clustered Regularly Interspaced Short Palindromic Repeats, has its roots in the microbial world. Bacteria utilize this system as a defense mechanism against viruses. Essentially, this natural process has been hijacked and optimized for gene editing in various organisms, harnessing its capabilities to precisely edit DNA sequences in a wide range of cell types.
Utilizing CRISPR involves two crucial components: the guide RNA (gRNA) that directs Cas9 to the specific location on the DNA, and the Cas9 enzyme itself, which introduces the double-strand breaks. Once the DNA is cut, the cell’s repair machinery can be directed to insert, delete, or alter the genetic material in the targeted location. This is where the term “knock-in” comes into play, signifying the insertion of new genetic material into a specific site of the genome.
The implications of CRISPR technology stretch far and wide, from agricultural enhancements to groundbreaking medical therapies. It is this versatility and precision that make CRISPR a phenomenal tool in the genetic toolkit, paving the way for innovative solutions to longstanding challenges.
Historical Context and Development
To fully grasp the significance of CRISPR-Cas9, it is crucial to look back at its developmental lineage. The discovery of the CRISPR-Cas system dates back to the late 1980s when scientists first identified these sequences in bacterial DNA. However, the transformation of this knowledge into a gene-editing tool took several more years.
In 2012, the CRISPR-Cas9 system was reworked by Jennifer Doudna and Emmanuelle Charpentier, who demonstrated its potential as a powerful method for genome editing. Their pioneering work laid the groundwork for what would become one of the most impactful technological advancements in genetic engineering. The rapid evolution of this technology has enabled a plethora of studies and experiments that push the boundaries of scientific understanding and application.
The Role of Cas9 in Genome Editing
Cas9, derived from the type II CRISPR system, is often singled out for its crucial role in genome editing. As a programmable endonuclease, it offers unmatched efficiency in creating double-strand breaks in DNA. Once Cas9 binds to the guide RNA and locates the target sequence, it creates a break in the DNA—this activates the cell's natural repair mechanisms, which are then manipulated to incorporate desired genetic material.
This capacity for targeted genome alterations is what allows researchers to knock in specific genes, creating cell lines that possess new functionalities. As a result, various research fields, including oncology, immunology, and regenerative medicine, have found immense value in employing Cas9 for practical applications.
"CRISPR-Cas9 not only helps scientists decode biological mysteries but also opens doors to therapeutic innovations previously thought impossible."
Understanding the Knock-In Process
The knock-in technique within gene editing is an innovative approach that has transformed genetic research and biotechnology. Understanding the knock-in process is fundamental in shaping precise genetic modifications that can lead to breakthroughs in various fields, including medicine, agriculture, and cell biology. The significance of the knock-in process lies in its ability to introduce specific genetic alterations or insertions into a desire location within an organism’s genome. This exactitude allows scientists to model diseases, study gene function, or develop new therapeutic strategies with unprecedented accuracy.
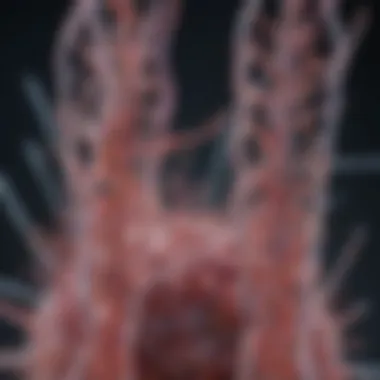
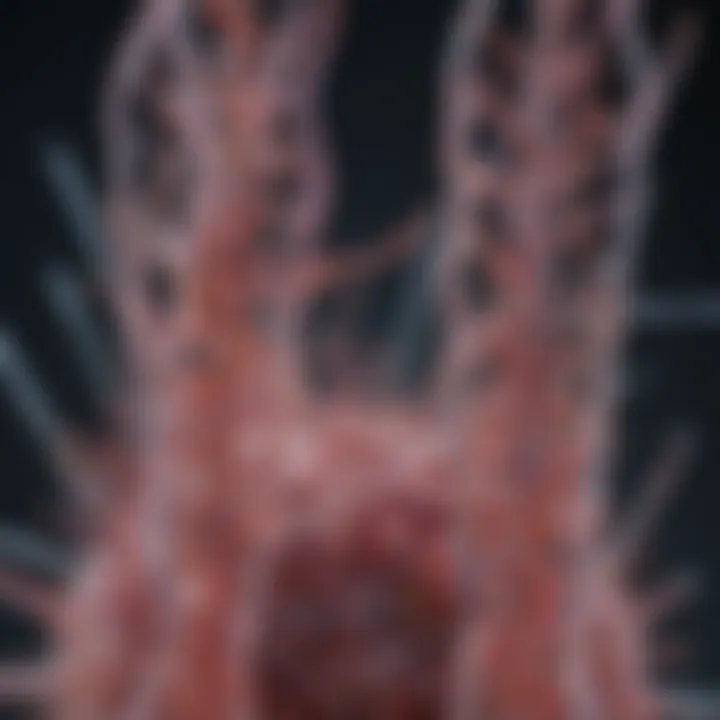
Mechanisms of Gene Insertion
At the heart of the knock-in process are the mechanisms that govern gene insertion. Here, the role of CRISPR-Cas9 is pivotal. This technology utilizes a guide RNA to direct the Cas9 enzyme to a precise spot in the genome. Once it arrives at the target site, Cas9 introduces a double-strand break (DSB). This break is a crucial first step, as it activates the cell's natural repair mechanisms.
The two primary pathways for DNA repair are homologous recombination and non-homologous end joining. In a knock-in setting, researchers exploit homologous recombination, where the cell uses an external donor template to repair the DSB.
Key factors at play include:
- Template Design: The donor template should ideally have homology arms at either end corresponding to sequences adjacent to the break site, which facilitates the correct insertion of the desired gene.
- Delivery Method: Various methods can be employed to introduce the CRISPR components and donor template into cells, including electroporation or viral vectors.
- Efficiency of Insertion: This can be influenced by cellular conditions, such as the cell cycle stage or the presence of other regulatory factors.
This intricate dance of molecular events underscores the necessity of a deep understanding of cellular mechanisms to optimize knock-in efficiency.
Designing Donor Templates
The design of donor templates is another layer of complexity in the knock-in process. A well-structured donor template is essential for successful gene insertion. Generally, donor templates come in two forms: single-stranded oligonucleotides (ssODN) or double-stranded DNA (dsDNA). Each serves its purpose based on the context of the gene editing task at hand.
When designing these templates, elements to consider include:
- Length and Composition: Homology arms typically range from 500 to 2,000 base pairs, and their length can significantly affect the efficiency of homologous recombination.
- Modification Types: The intended genetic alteration must be meticulously incorporated into the donor template, whether it’s a simple nucleotide substitution or a larger insertion containing a reporter gene.
- Potential Off-Target Effects: Care needs to be taken to ensure the designed template does not have sequences similar to unintended genomic locations, which could lead to off-target insertions.
Advancements in computational tools and algorithms now aid researchers in designing optimal donor templates that maximize specificity and efficiency while minimizing unintentional errors. These innovative strategies continue to push the boundaries of what is achievable with CRISPR-Cas9 technology, setting the stage for future discoveries and applications in genome editing.
"In the realm of gene editing, precision is not just a goal; it's a requirement for success. Every base pair counts in the intricate story of life."
Cell Line Models and Their Importance
Cell line models play a crucial role in the realm of genetic research, particularly when we talk about advanced techniques like CRISPR-Cas9 knock-in technology. They serve as a platform for scientists to manipulate genes and observe the outcomes of these modifications in a controlled environment. This investigative approach allows researchers to unravel complexities of various biological processes and their interactions without the ethical quandaries of working with whole organisms.
The significance of utilizing cell line models can be dissected into several core aspects:
- Controlled Environment: Unlike in vivo models, cell lines provide an opportunity to isolate variables. This isolation helps in studying the precise effects of gene alterations.
- Reproducibility: Once a cell line is established, it can be standardized across multiple experiments, enhancing the reproducibility of the results.
- Scalability: Cell lines can easily be grown in larger quantities, facilitating high-throughput experiments that are impractical with other models.
In terms of specific types of cell lines, numerous varieties are utilized to address a broad spectrum of research questions. Understanding the different types will illuminate how they fit the specific needs within the context of CRISPR knock-in research.
Types of Cell Lines Used in Research
In the pursuit of knowledge, researchers have access to a multitude of cell lines, each with its own characteristics suited for various applications. Some of the most common ones include:
- HeLa Cells: Derived from cervical cancer, these are one of the oldest and most widely used immortal cell lines. They are invaluable for studying cellular biology, cancer research, and virology.
- 293T Cells: Frequently used for virus production and gene expression studies due to their high transfection efficiency.
- iPSCs (Induced Pluripotent Stem Cells): Created from adult cells, these can differentiate into various cell types, making them a powerful tool for disease modeling and regenerative medicine.
Each type of cell line has its advantages, and choosing the right model is essential for the specific question at hand. This selection can significantly impact the results and interpretations gleaned from experiments involving CRISPR-Cas9 technology.
Advantages of Knock-In Cell Lines
The application of knock-in techniques in cell lines carries a host of advantages:
- Precision: Knock-in cell lines allow for precise modifications at targeted genomic locations, allowing researchers to study the effects of specific genes or mutations.
- Functional Studies: They enable functional analysis of gene expression and the role of particular proteins in disease processes.
- Translatability: These models help bridge the gap between basic research and clinical applications, refining therapeutic strategies aimed at genetic disorders.
Moreover, the adaptability of knock-in cell lines fosters innovation in experimental design, nurturing the next wave of biological discoveries.
Challenges in Developing Knock-In Cell Lines
While the promise of knock-in technology is substantial, the journey to developing these cell lines is not without its hurdles. Some of the challenges include:
- Low Efficiency: The knock-in process may suffer from relatively low efficiency, necessitating rigorous selection of successfully edited cells.
- Off-target Effects: There’s always a risk of unintended mutations elsewhere in the genome, which complicates the interpretation of results.
- Ethical and Regulatory Concerns: Researchers must navigate a complex landscape of ethical issues and regulatory standards, particularly given the implications of genetic editing technologies.
Despite these challenges, the combined advantages and persistent research efforts in improving methodologies continue to pave the way for breakthroughs using CRISPR-Cas9 knock-in strategies.
"Cell line models are like mirrors reflecting the genetic landscape, helping us better understand our complexities in health and disease."
Therefore, gaining a solid grasp of these aspects is fundamental for anyone looking to engage with the intensively evolving field of genetic research. As we proceed, the applications of CRISPR-Cas9 knock-in technology will come into clearer focus.
Applications of CRISPR-Cas9 Knock-In Technology
The applications of CRISPR-Cas9 knock-in technology are vast and varied, playing a pivotal role in the realm of genetic research. This section delves into how this innovative tool is transforming functional genomics, enabling precise modeling of genetic diseases, and paving the way for therapeutic advancements in drug development. The importance of these applications lies not only in their scientific merit but also in their potential to impact human health and disease management.
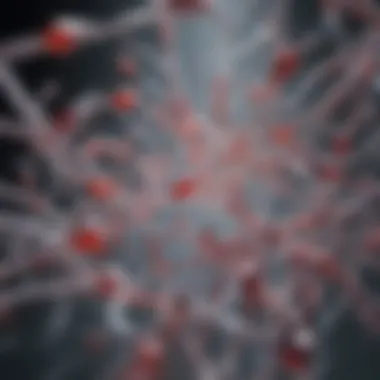
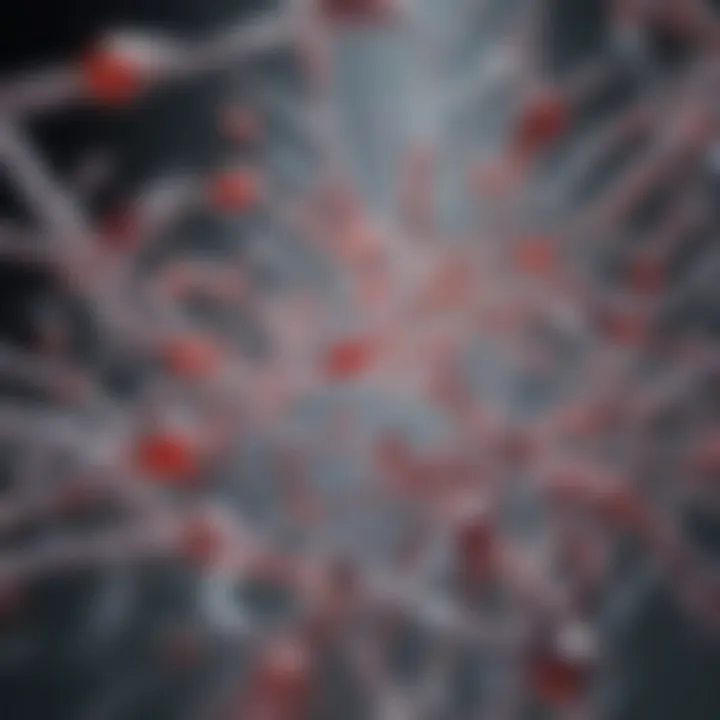
Functional Genomics
Functional genomics involves understanding the complex roles that genes and their variants play in biological systems. CRISPR-Cas9 knock-in technology serves as a powerful lens through which researchers can scrutinize gene function by inserting specific genetic elements into the genome. This allows for the direct observation of phenotypic changes arising from particular gene modifications.
Aligning the specific uses of this technology with the goals of functional genomics, it enables:
- Targeted Gene Studies: Inserting reporter genes can highlight the expression patterns of certain genes in various conditions or during specific developmental stages.
- Gene Interaction Exploration: Understanding relationships between multiple genes helps in elucidating pathways critical to cellular functions.
- Drug Target Validation: By creating cell lines with specific genetic alterations, researchers can assess how these changes affect drug responses, aiding in the identification of potential therapeutic targets.
Such insights foster a deeper understanding of cellular machinery and its regulation, ultimately leading to breakthroughs in treating diseases at their genetic roots.
Modeling Genetic Diseases
The modeling of genetic diseases has become significantly more refined thanks to CRISPR-Cas9 knock-in techniques. This technology allows scientists to emulate the genetic conditions seen in human populations within model organisms like mice and cell lines. By accurately replicating mutations that cause specific disorders, researchers can create more reliable models for studying pathophysiology and testing new treatments.
Key aspects of disease modeling include:
- Replicating Human Mutations: By inserting human disease-causing alleles, models can reproduce crucial aspects of diseases like cystic fibrosis or Huntington's disease.
- Studying Disease Mechanisms: Through these models, researchers can investigate how specific genetic alterations impact cellular function and contribute to disease progression.
- Therapeutic Testing: Testing potential therapies on these models can provide immediate feedback on efficacy and safety, expediting the translational research process.
With these models, the once daunting task of studying complex diseases becomes more manageable, leading to more effective treatment strategies.
Therapeutic Potential and Drug Development
The therapeutic applications of CRISPR-Cas9 knock-in technology are particularly promising. By making precise corrections to genetic faults, this technology opens the door to gene therapy—offering hope for previously untreatable diseases. It represents a shift from merely managing symptoms of genetic disorders to potentially correcting the root causes.
In the context of drug development, CRISPR-Cas9 further enhances the landscape by:
- Identifying Drug Targets: Knowing how specific genes contribute to diseases allows researchers to pinpoint where therapies should focus.
- Screening Compounds: Innovative screening methods can be used to identify compounds that effectively modulate targets established through knock-in studies.
- Personalized Medicine: As genomic information becomes more accessible, tailoring therapies based on an individual’s genetic profile moves closer to reality, improving treatment outcomes.
Evidence of this potential is seen in diverse fields, from oncology to inherited genetic disorders, showcasing how CRISPR's adaptability might reshape the future of medicine.
"The power of CRISPR technology lies not only in its precision but in the doors it opens for potential solutions to some of the toughest challenges in genetics."
Methodologies for Creating Knock-In Cell Lines
The creation of knock-in cell lines is a cornerstone in the deployment of CRISPR-Cas9 technology. This methodology encompasses an array of techniques and strategies necessary to accurately insert genetic material into specific locations within the genome. Understanding the strategies for creating knock-in models is crucial for researchers aiming to explore gene function and develop potential therapies. By navigating this landscape deftly, researchers can not only achieve precise genetic modifications but also glean insights into complex biological processes relevant to health and disease.
Gene Editing Protocols
At the heart of knock-in cell line development are the gene editing protocols that researchers meticulously follow. The first step often involves designing an appropriate guide RNA (gRNA) that directs the Cas9 enzyme to the target genomic location. This is no small feat; the specificity of the chosen gRNA directly impacts the efficiency of insertion.
After gRNA design, the next step typically consists of delivering the Cas9 protein along with the gRNA and a donor template into the target cells. Generally, a plasmid carrying the desired sequence to be knocked in, alongside appropriate homology arms, is critical here. These homology arms allow for homologous recombination, a natural process that cells use to repair double-strand breaks.
An essential consideration in these protocols is the transfection method: researchers might choose between electroporation, lipofection, or viral vectors, each with its own pros and cons. Electroporation, for instance, offers high efficiency but can cause cellular stress, while viral vectors may provide a gentler approach but often have limitations regarding the size of the genetic payload.
Additionally, optimizing conditions such as temperature, incubation time, and concentration of reagents can significantly influence the outcome.
Here’s a simplified overview of the typical workflow:
- Designing gRNA
- Preparing donor template
- Selecting delivery method
- Transfecting target cells
- Incubating for homology-directed repair
The precision of these protocols, combined with a well-timed approach, ultimately governs the success of knock-in cell line generation.
Selection and Screening Strategies
Once the gene editing protocols have been executed, the next hurdle involves the selection and screening of successful edits. This process can feel like searching for a needle in a haystack, given the probability of off-target effects or unedited alleles.
To identify successful knock-ins, several strategies can be employed.
- Antibiotic Selection
By including a selectable marker in the donor template, researchers can take advantage of antibiotic resistance as a selection criterion. Only those cells that incorporate the desired sequence survive, simplifying the screening process. - PCR Screening
Using polymerase chain reaction (PCR), researchers can amplify the target region to confirm the presence of the knock-in sequence. The size of the PCR product can differ from that of an unedited cell, offering a reliable method for validation. - Sanger Sequencing
Following PCR, Sanger sequencing provides a definitive confirmation of successful edits by deciphering the exact nucleotide sequence. This step can be somewhat arduous, yet it ensures accuracy. - Next-Generation Sequencing (NGS)
For a more comprehensive analysis, NGS can reveal off-target effects and other unintended edits across the genome. This may provide a broader understanding of the edits, offering more confidence in the cellular model.
In this selection and screening phase, researchers should also consider polarizing their approach based on the goals of their study—whether they require a quantitative overview or a deep dive into specific modifications.
Overall, the success of developing knock-in cell lines hinges on the meticulous structuring of both gene editing protocols and the preemptive planning of selection strategies. Through careful execution of these methodologies, researchers can push the boundaries of what is currently understood about genetic research, ultimately leading to innovative applications that could benefit both science and society.
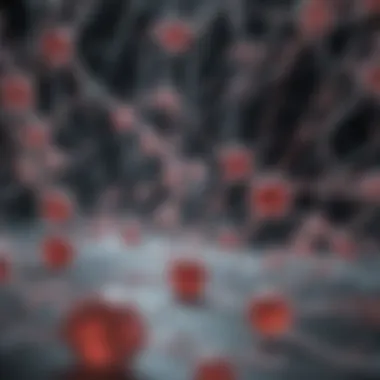
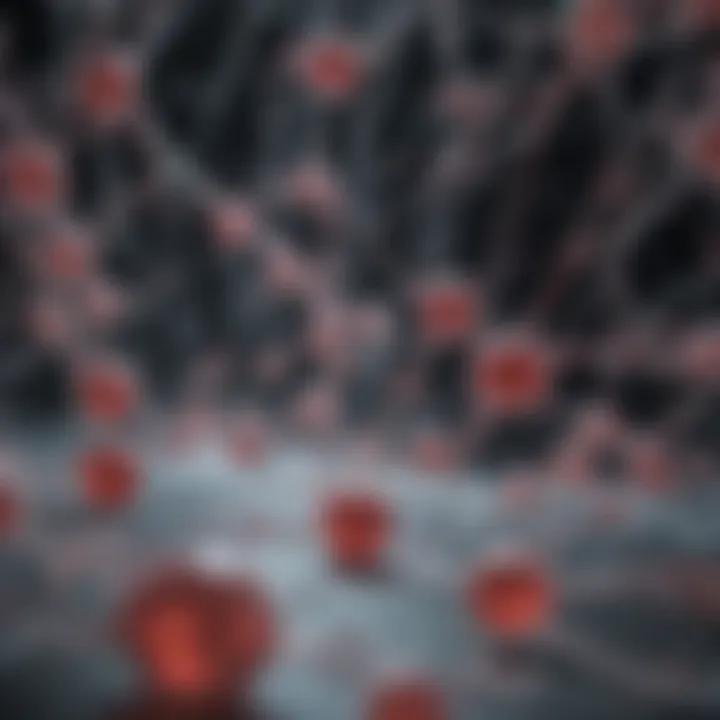
Ethical Considerations in Gene Editing
The topic of ethical considerations in gene editing has become increasingly prominent in the conversation surrounding CRISPR-Cas9 technology. As we tread deeper into uncharted territory of genetic manipulation, it is essential to consider the moral implications that accompany scientific advancements. CRISPR not only provides a powerful tool for gene editing but also raises significant questions about the limits of human intervention in nature, the potential for misuse, and the societal consequences of genetic modifications.
- Importance of Ethical Considerations
The significance of ethical evaluations in gene editing cannot be understated. The potential for unintended consequences can lead to irreversible ecological misbalances or health impacts. For instance, genes edited in one organism may have unforeseen downstream effects in ecosystems or even in human populations. By carefully weighing these ethical dimensions, researchers can ensure responsible development and application of CRISPR technology. - Specific Benefits and Concerns
While CRISPR-Cas9 offers many benefits, such as the chance to eradicate genetic diseases and improve food security, it also poses specific ethical dilemmas:
- Human Enhancement vs. Therapy: The distinction between therapeutic applications and enhancement raises questions about equity and access. Who gets the benefits of gene editing? The rich or everyone?
- Germline Editing: Editing genes that are passed on to future generations could cause permanent changes in the gene pool, and if done irresponsibly, it could lead to unforeseen consequences for humanity.
"Without ethical guidelines, we face a risk of acting without foresight, potentially endangering ourselves and future generations."
Navigating these considerations is vital if society is to harness CRISPR-Cas9 technology securely and ethically.
Regulatory Frameworks and Guidelines
The regulatory landscape for gene editing is both complex and dynamic. It reflects the various national perspectives on bioethics and the extent to which lawmakers seek to control scientific advancements. Countries vary significantly in their approaches; for example, some nations have strict prohibitions on germline editing, while others are more permissive.
Key Elements of Regulatory Frameworks:
- Safety Assessments: Regulations often include mandatory assessments of potential risks associated with gene editing in clinical trials for safety and efficacy.
- Public Consultation: Engaging the public in discussions about gene editing principles must be prioritized. This fosters wider acceptance and understanding of the ethical nuances.
- International Cooperation: Biological research, especially creating genetic modifications, knows no borders. Thus, international guidelines and collaboration are essential for establishing consensus on ethical boundaries.
Public Perception and Societal Impact
The societal impact of gene editing, particularly through CRISPR-Cas9, hinges upon public perception. As more information emerges, public sentiment can shift dramatically, influencing policies and research funding. Understanding how the general populace views gene editing is crucial for scientific advancement and societal harmony.
Factors Influencing Public Perception:
- Media Representation: The portrayal of gene editing technologies in media plays a pivotal role. Negative depictions tend to engender fear, complicating progressive scientific efforts. Conversely, showcasing successful applications can breed hope and acceptance.
- Cultural Influences: Cultural beliefs and values drastically influence how different communities accept or reject gene editing. For example, some societies may view the manipulation of genes as a violation of natural processes, while others may embrace it as a necessary evolution of medicine.
- Education and Awareness: Promoting broader understanding of genetic tools and their potential impacts fosters a more informed populace. Educational initiatives can arm individuals with essential knowledge about the risks and benefits of gene editing, allowing for more balanced perspectives.
Recent Advances and Future Directions
The field of CRISPR-Cas9 technology is advancing rapidly, continually evolving and expanding our capabilities in gene editing. These recent advances are not merely incremental; they signify a sort of paradigm shift in how researchers can approach gene-related challenges. Analyzing the latest innovations and anticipated trends in genetic research can provide insight into future possibilities, such as personalized medicine, agricultural resilience, and treatments for genetic disorders.
Innovations in CRISPR Technology
In recent years, the breakthroughs surrounding CRISPR technology are numerous and noteworthy. For instance, newer variants of the Cas9 protein, such as Cpf1 (also known as Cas12a), have emerged. Cpf1 differs from the traditional Cas9 in its cutting mechanism, which allows for a more precise targeting of genes with a simpler design, often leading to decreased off-target effects. This advancement has opened new doors for applications in both therapeutic settings and agricultural modifications.
Another significant stride is the development of prime editing. Dubbed as a "search-and-replace" tool for DNA, prime editing enables researchers to make precise changes to the genome without causing double-strand breaks. This method is touted for its flexibility and accuracy, offering a high degree of control, which is crucial for applications that require exact genome editing to avoid unintended consequences.
Furthermore, the rise of multiplexing strategies allows for the simultaneous targeting of multiple genes. This capability could be a game changer, especially in the area of complex genetic diseases where multiple loci may need editing in tandem to fully understand and address the conditions. For example, a study leveraging multiplexed CRISPR techniques reported significant progress in engineering plants with enhanced traits, hinting at an exciting future in agricultural biotech.
"Innovations like prime editing not only enhance precision but also reduce potential off-target effects, setting a new standard for genetic modification techniques."
Upcoming Trends in Genetic Research
Looking forward, the trends are likely to reflect a blend of technological advancement and a growing understanding of ethical considerations surrounding gene editing. The integration of artificial intelligence in bioinformatics stands to change the landscape dramatically. By utilizing large datasets and sophisticated algorithms, scientists can now better predict outcomes of CRISPR interventions and streamline the design process of sgRNAs or donor templates.
Moreover, the convergence of CRISPR technology with other areas of genomics and biotechnology, like single-cell sequencing, is gaining traction. This combination is likely to provide intricate insights into gene interactions and the impact of gene editing at a cellular level, potentially leading to breakthroughs in understanding diseases that arise from complex genetic interactions.
Another noteworthy trend is the shift toward in vivo applications. While much of the current work has been in vitro, the push is on to develop safe and effective methods for delivering CRISPR components into living organisms. This area is essential for the eventual realization of gene therapies that can treat inherited disorders directly within the body.
In summary, the advances in CRISPR technology and the emerging trends in genetic research showcase promising directions that could reshape the future of medicine and agriculture. The ability to edit genomes with high precision opens up avenues for innovations that were once the stuff of science fiction. As the research community continues to push boundaries, keeping an eye on these developments will be crucial for understanding and harnessing the full potential of CRISPR-Cas9 technologies.
Epilogue
The conclusion of this article brings together the threads of understanding woven through the exploration of CRISPR-Cas9 knock-in technology. Recognizing this technology's impact is crucial for students, researchers, and professionals who are deeply embedded in genetic research, biotechnology, and beyond. CRISPR-Cas9 is not merely a tool for scientists; it embodies a paradigm shift in how we approach gene editing and cellular biology.
Summarizing the Impact of CRISPR-Cas9
CRISPR-Cas9 has revolutionized the field of genetic engineering, allowing for precise modifications in the genetic code. The ability to perform knock-in operations enables researchers to insert genes of interest into specific loci within the genome. This offers several significant benefits:
- Precision and Efficiency: Compared to older technologies like TALEN or ZFN, CRISPR-Cas9 allows for quicker and more accurate gene alterations without the risk of unintended mutations.
- Broad Applicability: The versatility of this technique means it can be applied across a plethora of organisms, from simple bacteria to more complex mammalian cells. This opens doors for advances in functional genomics and therapeutic applications.
- Baseline for Future Research: By summarizing the findings and pathways elucidated by CRISPR work, scientists can build on existing knowledge. As researchers further understand gene functions, the implications of their tools expand, fostering innovation.
"The ramifications of CRISPR technology extend beyond laboratory research, resonating with ethical, societal, and personal dimensions of the future of medicine."
Looking Ahead: The Future of Gene Editing
Looking forward, the future of gene editing, particularly through CRISPR-Cas9, appears promising but also complex. Future directions could include:
- Enhanced Specificity: As the search for improved accuracy continues, the development of next-generation CRISPR systems aims to mitigate off-target effects, ensuring that the modifications target only specified genes without unintended consequences.
- Regenerative Medicine: The potential applications for CRISPR in regenerative medicine are vast. Researchers envision using knock-in techniques to correct genetic disorders and to apply therapies that may one day lead to the restoration of normal function in affected tissues.
- Synthetic Biology: The intersection of CRISPR technology and synthetic biology can give rise to novel applications such as engineered living systems capable of complex tasks. This could transform biotechnology and lead to solutions for global challenges.
As we navigate the complexities ahead, the understanding of CRISPR-Cas9 and its implications cannot be overstated. It demands thoughtful consideration and ethical oversight to harness its full potential responsibly. By grasping the advancements and challenges, we position ourselves at the forefront of a genetic engineering revolution that could redefine the fabric of life itself.