Deciphering the Complex Structure of DNA
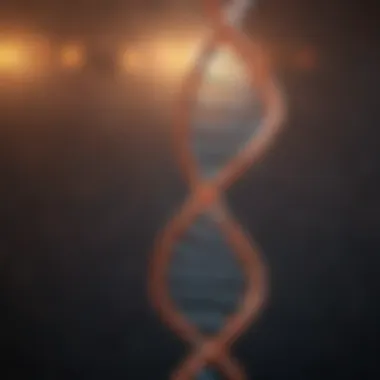
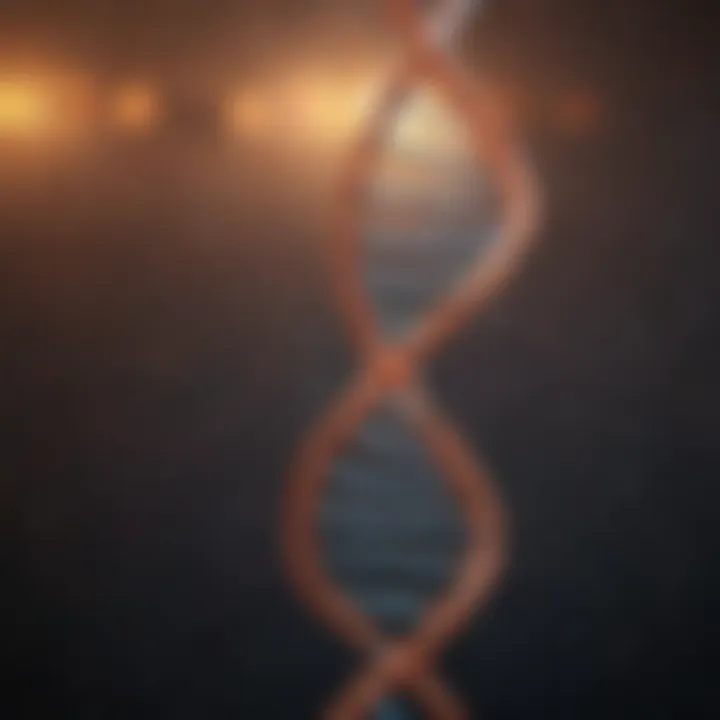
Intro
DNA, or deoxyribonucleic acid, serves as the blueprint of life, governing the development and functioning of all known organisms. Understanding the components of DNA is essential for students, researchers, educators, and professionals in the fields of biology and genetics. This article delves into the intricate structure of DNA, focusing on the fundamental elements and processes that define this critical biomolecule. It is imperative not only to grasp the basic building blocks of DNA but also to appreciate their complex interactions and roles in the broader context of molecular biology.
Research Overview
Key Findings
In examining DNA, several key points have emerged:
- Structure: DNA comprises two long strands forming a double helix.
- Nucleotides: The basic units of DNA are nucleotides, consisting of a sugar, a phosphate group, and a nitrogenous base.
- Base Pairing: The nitrogenous bases—adenine, thymine, cytosine, and guanine—pair specifically: adenine with thymine and cytosine with guanine.
- Genetic Information: The sequence of these bases encodes genetic information, crucial for the synthesis of proteins.
This synthesis is a foundational process impacting various biological functions and research applications.
Study Methodology
The exploration of DNA structure has evolved through various methodologies. Key approaches include:
- X-ray Crystallography: This technique was instrumental in determining the double helix structure of DNA. It revealed how closely packed the molecules are and how they interact spatially.
- Nucleic Acid Sequencing: Advancements in sequencing technologies have allowed for detailed mapping of DNA sequences. Techniques like next-generation sequencing have revolutionized genetic research, providing insights into genomic diversity.
- Bioinformatics: The integration of computational tools helps in analyzing complex data derived from DNA studies. These technologies enable researchers to draw meaningful conclusions from large datasets.
Background and Context
Historical Background
The discovery of DNA's structure is a monumental event in scientific history. In 1953, James Watson and Francis Crick proposed the double helix model based on Rosalind Franklin's X-ray diffraction images. This breakthrough has led to an explosion of research aimed at understanding the mechanisms of heredity and the role of DNA in evolution.
Current Trends in the Field
Today, the study of DNA remains a vibrant area of research. Some current trends include:
- Genomic Editing: Techniques such as CRISPR-Cas9 have emerged, allowing precise modifications in DNA, paving the way for potential treatments of genetic disorders.
- Synthetic Biology: This field focuses on designing and constructing new biological parts, devices, and systems, driven by a deeper understanding of DNA structure and function.
- Personalized Medicine: There is an increasing emphasis on utilizing genetic information for tailored medical interventions, particularly in cancer treatment and rare genetic conditions.
“The understanding of DNA not only defines the essence of life but also opens doors to innovative solutions in medicine and biology.”
With a solid grounding in the components of DNA, readers can appreciate its role not just in biology but in technology and medicine, forming the basis for continued scientific advancement.
Prologue to DNA
Understanding DNA is essential for comprehending the biological processes that govern life. DNA, or deoxyribonucleic acid, serves as the blueprint for all living organisms. By unraveling its structure and components, we gain insight into how genetic information is stored, transmitted, and utilized in various biological functions.
Historical Context
The study of DNA has evolved significantly since its discovery. In the late 19th century, Friedrich Miescher first isolated what he termed 'nuclein' from white blood cells. This marked the foundation of molecular biology. However, it was not until the 1950s that James Watson and Francis Crick proposed the double helix model of DNA. Their work, built upon research by Rosalind Franklin and Maurice Wilkins, provided a clear visual structure of DNA, paving the way for advancements in genetic research.
This historical backdrop is crucial for appreciating the contemporary implications of DNA studies. It highlights the collaborative nature of scientific discovery and the gradual accumulation of knowledge that has led to our current understanding of genetic material.
Importance of DNA in Biology
DNA holds a central role in biology, acting as the repository of genetic information. It carries instructions for the development, functioning, growth, and reproduction of all known organisms and many viruses. Some key points to consider:
- Genetic Blueprint: DNA sequences dictate the synthesis of proteins, the workhorses of the cell, which perform a wide range of functions.
- Inheritance: DNA is passed from parents to offspring, ensuring continuity of genetic traits. This is a fundamental concept in genetics, allowing for the study of heredity.
- Molecular Mechanisms: The understanding of DNA underpins crucial processes such as gene expression and regulation, essential for development and response to environmental changes.
By engaging with the components and structure of DNA, researchers and students in the field can open doors to innovative studies in genetics, healthcare, and biotechnology.
"DNA is not just a molecule; it is the foundation of life itself…"
Understanding its complexities and implications is vital for future innovations in genetic research and beyond.
Structural Components of DNA
The structural components of DNA are fundamental to its function and significance in biological systems. Understanding these components provides a clearer view of how genetic information is stored, replicated, and expressed within organisms. The three primary elements include nucleotides, phosphate groups, and deoxyribose sugar. Each plays a critical role in the stability and functionality of DNA, ensuring that genetic material is preserved accurately through generations.
Nucleotides
Nucleotides are the building blocks of DNA. Each nucleotide consists of three parts: a nitrogenous base, a phosphate group, and a deoxyribose sugar. The arrangement and pairing of these nucleotides create the genetic sequence, which dictates organismal characteristics. In total, there are four types of nitrogenous bases in DNA: adenine, guanine, cytosine, and thymine. Each base interacts with others in specific ways, contributing to the overall structure and function of DNA.
Adenine
Adenine, one of the four nitrogenous bases, pairs exclusively with thymine in DNA. Its presence is crucial for the formation of base pairs, which stabilize the DNA structure. A key characteristic of adenine is its ability to form double hydrogen bonds with thymine, establishing a robust connection essential for the integrity of the DNA double helix. Adenine is beneficial in this discussion because it exemplifies the specificity of base pairing that underlies genetic fidelity. A unique feature of adenine is that it can also play roles in energy transfer in biological systems, as seen in ATP. This dual functionality highlights its importance in both structural and energetic contexts. However, its over-representation in certain sequences can lead to mutations.
Guanine
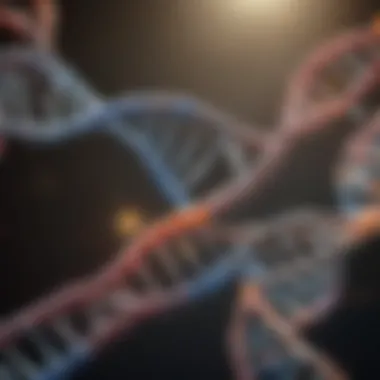
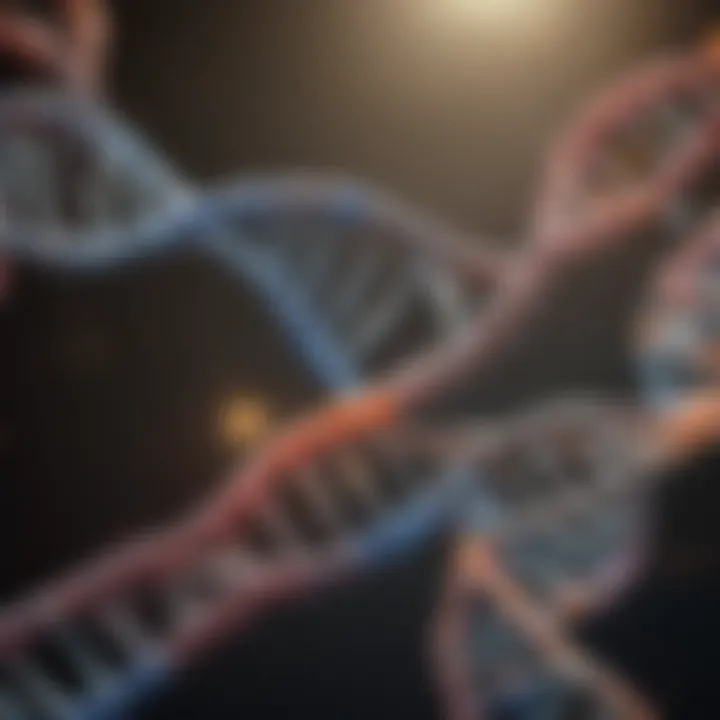
Guanine, another critical nitrogenous base, pairs specifically with cytosine. Its significance lies in the triple hydrogen bonds it forms with cytosine, offering heightened stability to the DNA structure. Guanine's key characteristic is its unique chemical structure, which includes a carbonyl group that participates in hydrogen bonding. In this article, guanine represents an essential contributor to the encoding of genetic information. One unique feature of guanine is its role in the synthesis of guanosine triphosphate (GTP), a crucial molecule in various cellular processes, including protein synthesis. While guanine is functionally vital, its susceptibility to oxidative damage can result in mutations, which could have implications for organismal health.
Cytosine
Cytosine pairs with guanine, forming part of the genetic code's backbone. Its primary significance in this discourse is its role in ensuring stability through its three hydrogen bonds with guanine. A key characteristic of cytosine is its ability to undergo deamination, a chemical reaction that can lead to point mutations if not repaired. Cytosine's unique feature is its involvement in the regulation of gene expression. Cytosine methylation is a common epigenetic modification impacting cellular processes. This advantage highlights cytosine's influence beyond mere structural roles; however, the potential for mutations following deamination poses risks to genomic integrity.
Thymine
Thymine, the last nitrogenous base in DNA, pairs with adenine. Its simplistic structure and ability to form double hydrogen bonds make thymine a reliable partner for adenine in maintaining the DNA structure. A key aspect of thymine is its role in protecting DNA from hydrolytic damage, thus contributing to the overall stability of the molecule. Thymine's benefit in this article lies in its representation of a commonly recognized base, connecting well with discussions of mutations. Thymine also offers unique features, such as lacking a hydroxyl group on the 5th carbon, distinguishing it from uracil in RNA. However, thymine dimers can form under UV radiation, leading to problematic mutations.
Phosphate Groups
Phosphate groups provide the linkage between nucleotides, forming the DNA backbone. Each phosphate connects to the 5' carbon of one sugar and the 3' carbon of another, creating a sugar-phosphate backbone that is crucial for DNA’s overall structural stability. This arrangement forms a polar molecule, with one end being the 5' terminus and the other the 3' terminus. The significance of phosphate groups in this article lies in their ability to facilitate structural integrity and charge properties, which are essential for interactions with histone proteins during DNA packaging.
Deoxyribose Sugar
Deoxyribose sugar is another critical component of DNA, contributing to the overall structure and functionality of the molecule. Unlike ribose in RNA, deoxyribose lacks an oxygen atom at the 2' position, giving DNA its name. The significance of deoxyribose lies in its stability, which is crucial for the long-term preservation of genetic information. Its key characteristic supports the formation of the double helix by forming linkages with phosphate groups and nitrogenous bases. Unique features of deoxyribose include its role in determining the directionality of the DNA strand. This aspect is vital for processes like DNA replication and transcription, where the orientation plays a vital role.
In summary, understanding these structural components of DNA is essential for appreciating the complexity of genetic information storage and transmission. Each element contributes to the stability, integrity, and functionality of DNA, underscoring the importance of this biomolecule in biological processes.
Double Helix Structure
The double helix structure of DNA is fundamental to understanding its function in biological systems. This arrangement not only determines how genetic information is stored but also influences how genes are expressed and regulated. The significance of the double helix is rooted in its stability and ability to replicate accurately. Without this specific configuration, the mechanisms that underpin genetic inheritance would not function as we observe today.
Discovery of the Helical Structure
The discovery of the helical structure of DNA is a landmark event in the field of molecular biology. In 1953, James Watson and Francis Crick presented their model of DNA as a double helix, an insight built upon data from Rosalind Franklin’s X-ray diffraction images. Their model demonstrated how two strands of DNA are intertwined, held together by base pairs that adhere to specific pairing rules. This structure effectively explains how genetic information is conserved and transmitted during cell division.
"The most remarkable aspect of the double helix is how it preserves genetic information while allowing for changes that drive evolution."
The model proposed by Watson and Crick provided a framework for subsequent research into genetic replication, mutation, and manipulation. Understanding how the helical structure resists physical force while maintaining accessibility for processes such as transcription is key to many biological applications.
Major and Minor Grooves
In the structure of the DNA double helix, two types of grooves are formed: the major groove and the minor groove. These grooves arise from the angles at which the strands wrap around each other. The major groove is larger and affords greater access to proteins and enzymes that interact with DNA. On the other hand, the minor groove is narrower, presenting a more limited space for interaction.
The grooves play a critical role in recognizing specific sequences of DNA. Proteins that bind to DNA, such as transcription factors, often do so through the major groove due to its accessibility. This ability to open one strand while maintaining the integrity of the other is vital for processes like gene expression and replication, where selective access to the genetic code is required.
In summary, the double helix structure provides both physical stability and chemical accessibility to the genetic material. The discovery of this arrangement has laid the groundwork for advancements in genetic research, allowing scientists to explore the intricacies of heredity and the biochemical mechanisms that govern life.
Base Pairing Rules
Base pairing rules are fundamental to understanding the structure and function of DNA. They specify how nucleotides pair with each other, forming the rungs of the helix. This pairing is crucial because it ensures the accurate replication of genetic information during cell division. Moreover, understanding these rules adds depth to our comprehension of heredity, mutation, and gene expression.
Complementary Base Pairing
In DNA, two strands twist around each other, held together by pairs of nitrogenous bases. These bases are complementary, meaning that they can only pair in specific combinations: Adenine pairs with Thymine, while Guanine pairs with Cytosine. This arrangement is not arbitrary; it is rooted in the chemical properties of the bases themselves. For instance, Adenine and Thymine form two hydrogen bonds, creating a stable yet flexible bond. Conversely, Guanine and Cytosine form three hydrogen bonds, resulting in a stronger interaction. This strength is vital for the integrity of DNA, especially during the processes of transcription and replication.
- Adenine (A) - Pairs only with Thymine (T).
- Thymine (T) - Pairs only with Adenine (A).
- Guanine (G) - Pairs only with Cytosine (C).
- Cytosine (C) - Pairs only with Guanine (G).
This specificity in base pairing has significant implications. It influences the three-dimensional shape of DNA, affecting how it is recognized and read by various cellular machinery. For example, genes are transcribed into RNA based on these specific sequences, illustrating the connection between structure and function in genetic expression.
Hydrogen Bonding
Hydrogen bonding plays a pivotal role in stabilizing the DNA structure. The base pairs are held together by these weak interactions, which allow the two strands to separate easily during replication and transcription. The strength of the hydrogen bonds between Guanine and Cytosine compared to those between Adenine and Thymine results in variations in the melting temperatures of DNA segments. Higher GC content generally leads to greater stability under heat.
Furthermore, hydrogen bonding is crucial for ensuring the fidelity of DNA replication. During synthesis, DNA polymerase relies on accurate base pairing to create a complementary strand. If pairing is incorrect, it can lead to mutations, which may have profound effects on organismal biology.
"Complementary base pairing is not just a mechanism; it is the cornerstone of genetic fidelity and diversity."
In summary, base pairing rules highlight the intricacies of DNA structure and function, showcasing the relationships that underlie genetic processes. The association between complementary bases and their hydrogen bonding significantly contributes to the stability and replication of genetic material, making them essential concepts in molecular biology.
DNA Replication
DNA replication is a fundamental process that ensures genetic material is accurately copied before cell division occurs. This is essential for maintaining the genetic continuity of organisms. The significance of DNA replication in genetics and molecular biology cannot be overstated. Through replication, each daughter cell receives an identical set of DNA, which is crucial for growth, repair, and reproduction in organisms.
Mechanisms of Replication
The mechanisms of DNA replication involve a series of well-orchestrated steps. Initially, the double helix unwinds to separate the two strands. This unwinding is critical as it provides the template needed to synthesize new complimentary strands. Following unwinding, DNA is copied in a semiconservative manner. This means that each new double helix consists of one original and one newly synthesized strand. This conserves half of the original DNA, ensuring reliability and reducing errors.
The process occurs in various phases: initiation, elongation, and termination. During initiation, the unwinding occurs, and specific initiating proteins help in starting the replication at the origin of replication. Each strand serves as a template for building the new complementary strands during elongation. Finally, in termination, replication stops at specific sites, completing the synthesis of new DNA strands.
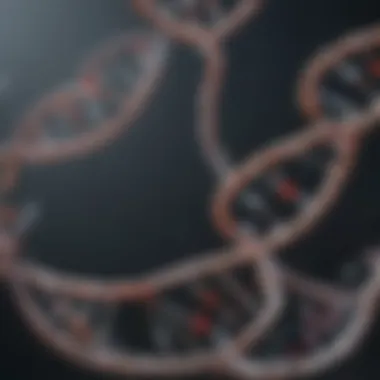
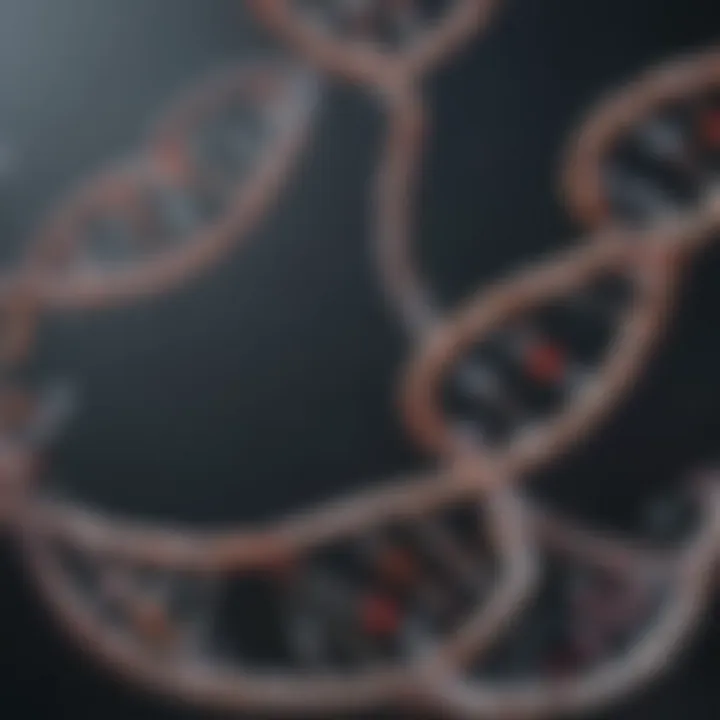
Role of Enzymes
DNA Polymerase
DNA Polymerase plays a crucial role in the replication process. Its primary function involves adding nucleotides to a growing DNA strand. This enzyme guarantees the accuracy of replication by proofreading and correcting errors during synthesis. A notable characteristic of DNA Polymerase is its ability to work only in one direction, which means it synthesizes DNA in a 5' to 3' direction. This directional synthesis is critical, as it influences how replication occurs on the leading and lagging strands.
DNA Polymerase is a preferred enzyme in various laboratory and clinical settings due to its high fidelity. The error-correcting ability ensures that the genetic information remains accurate, which is paramount in research involving DNA amplification or sequencing. However, one disadvantage is its inability to initiate new DNA strands. It requires a short RNA primer to add nucleotides, making it dependent on other factors in the replication process.
Ligase
Ligase is another essential enzyme in DNA replication. Its primary role is to join together the Okazaki fragments created during replication on the lagging strand. This enzyme effectively seals nicks in the sugar-phosphate backbone of the DNA, ensuring a continuous DNA strand. A key characteristic of Ligase is its specificity for DNA, requiring a different form of the enzyme for RNA.
The contribution of Ligase to the overall replication process is significant; without it, the lagging strand would remain fragmented, leading to incomplete genetic material. Despite its importance, Ligase's functioning can be featured by low efficiency in certain conditions, like high toxicity environments, which may hinder DNA repair processes.
Helicase
Helicase is critical for unwinding the double helix before DNA replication can begin. Helicase breaks the hydrogen bonds between complementary bases, allowing the two strands to separate. A characteristic feature of Helicase is its use of ATP (adenosine triphosphate) to fuel the unwinding of DNA. This enzymatic activity lays the groundwork for the new strands to be synthesized.
The unique advantage of Helicase is its speed in unwinding DNA, allowing for highly efficient replication. It ensures that replication can occur rapidly during cell division. However, a drawback of Helicase is its vulnerability to mutations, which can disrupt the replication fork and lead to potential cellular malfunction.
Understanding the roles of these enzymes is pivotal in the field of molecular biology. Their functions underline the complexity and precision required for accurate DNA replication.
Functional Aspects of DNA
The functional aspects of DNA encompass the various biological processes that this molecule performs, illustrating its critical role in living organisms. Understanding these functions is paramount for those studying genetics, molecular biology, and biochemistry. Key elements include gene expression and regulatory sequences, both of which dictate how genetic information is utilized within the cell. Their exploration reveals not only the complexity of genetic mechanisms but also the implications for evolutionary biology and biotechnology.
Gene Expression
Transcription
Transcription is a fundamental process in the gene expression pathway. This mechanism converts DNA into messenger RNA (mRNA), marking the first step in synthesizing proteins. It is characterized by the action of RNA polymerase, an enzyme responsible for reading the template DNA strand and constructing the complementary RNA strand. The significance of transcription lies in its capacity to express genes in a regulated manner, which allows cells to respond to their environment and engage in specialized functions.
A critical feature of transcription is its ability to produce multiple mRNA copies from a single gene, thereby amplifying the genetic message. This quality is beneficial, as it ensures a sufficient supply of proteins, even from a limited number of gene copies. However, transcription can also introduce variability, as it may lead to the inclusion of non-coding regions in mRNA, known as introns, which must be removed during RNA processing. Overall, this process ensures the effective flow of genetic information, acting as a linchpin in cellular operations.
Translation
Translation follows transcription and is equally crucial for gene expression. This process involves decoding the mRNA sequence to synthesize proteins, effectively translating genetic languages into functional molecules. Ribosomes, along with transfer RNA (tRNA), play essential roles in this intricate mechanism. The ribosome facilitates the assembly of amino acids into polypeptides, creating proteins that perform various functions in the organism.
A key characteristic of translation is its specificity, as each codon in the mRNA corresponds to a specific amino acid. This specificity is vital for maintaining the amino acid sequence's fidelity, ensuring that proteins are produced accurately according to their genetic instructions. The unique feature of translation includes its role in post-translational modifications, where proteins undergo further changes that can affect their function and activity. While translation is generally efficient, errors in this process can lead to the production of dysfunctional proteins, highlighting a disadvantage that must be managed within cellular systems.
Regulatory Sequences
Regulatory sequences are segments of DNA that play crucial roles in regulating gene expression. These sequences determine when, where, and how genes are expressed, acting much like switches that control the transcriptional activity of genes. Regulatory elements can include promoters, enhancers, and silencers. Each affects transcription in different manners, providing layers of control over gene expression.
Promoters are located near the beginning of genes and serve as binding sites for RNA polymerase and other necessary transcription factors. Enhancers can be positioned far from their target genes but still raise expression levels by helping to recruit the transcription machinery. Conversely, silencers diminish transcription levels of specific genes, capable of preventing RNA polymerase access.
Through the interaction of these regulatory elements, cells can finely tune gene expression in response to internal and external signals. This regulation is essential for development, cellular differentiation, and responses to environmental changes, all of which have profound implications in fields like cancer biology and genetic engineering. In summary, regulatory sequences fundamentally shape the functional landscape of DNA, contributing to the intricate web of life by fine-tuning gene activity.
Genetic Code
The genetic code serves as the blueprint for life. It is the set of rules by which the information encoded within genetic material is translated into proteins, which are vital for various biological functions. Understanding the genetic code is essential in biology, genetics, and biochemistry. It has implications in fields ranging from medicine to biotechnology. The intricate relationships between codons, amino acids, and their interpretations by the cellular machinery reveal the underlying complexity of genetic expression.
Codons and Amino Acids
The genetic code consists of sequences of nucleotides grouped in threes, known as codons. Each codon corresponds to a specific amino acid or a stop signal during protein synthesis. There are 64 possible codons, but only 20 standard amino acids. This redundancy is a critical aspect of the genetic code, allowing for variations in DNA sequences without altering the resultant proteins significantly.
For instance, the codons AUG and GUG both encode the amino acid methionine under certain conditions. This redundancy helps in minimizing the impact of mutations. In summary, the relationship between codons and amino acids is fundamental in comprehending protein synthesis and genetic encoding.
Degeneracy of the Code
The concept of degeneracy refers to the property where multiple codons can encode the same amino acid. This phenomenon plays a crucial role in providing a buffer against the deleterious effects of mutations. For example, the amino acid leucine can be encoded by six different codons: UUA, UUG, CUU, CUC, CUA, and CUG.
Thus, if a mutation occurs in the DNA sequence that changes one codon into another, there is a chance that the same amino acid will still be produced. This degeneracy ensures that the evolutionary process continues without incurring significant risk to protein function.
The presence of degeneracy in the genetic code adds a layer of resilience against genetic mutations, highlighting the elegance of biological systems.
Understanding degeneracy can aid researchers in gene editing and synthetic biology. It informs strategies for designing more effective interventions when correcting genetic disorders. Furthermore, studying the genetic code's redundancy leads to deeper insights into evolutionary mechanisms and adaptation strategies.
Mutations and DNA Repair
Mutations and DNA repair mechanisms play a critical role in genetics and molecular biology. They are essential for preserving the integrity of genetic information. Mutations are changes in the DNA sequence that can lead to variations in gene function and expression. This topic is significant because mutations can have both beneficial and detrimental effects on organisms. Understanding how mutations occur and how DNA repair mechanisms work is essential for comprehending the broader implications of DNA in life.
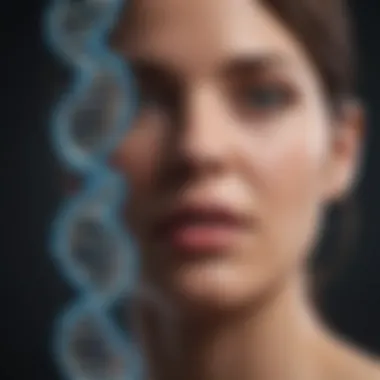
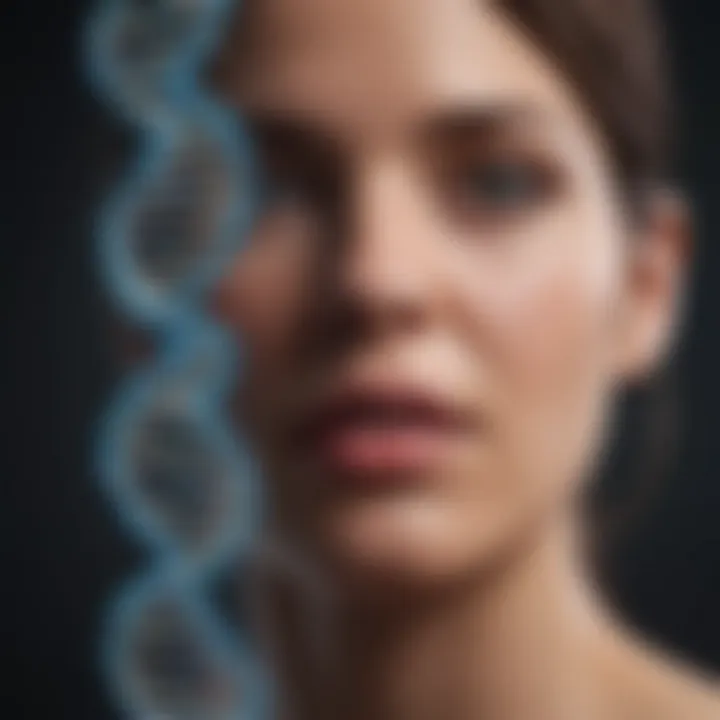
Types of Mutations
Point Mutations
Point mutations are specific alterations within a single nucleotide base pair in the DNA sequence. These mutations can lead to significant effects, such as amino acid changes in proteins. They are important to discuss because they are a common type of mutation affecting organisms.
One key characteristic of point mutations is that they include three main types: silent, missense, and nonsense mutations. Silent mutations do not change the amino acid sequence, while missense mutations lead to a different amino acid, possibly altering protein function. Nonsense mutations create a stop codon, resulting in premature termination of protein synthesis.
Point mutations are beneficial to include in this article due to their well-studied nature. They serve as prime examples for elucidating broader concepts of genetic variability. A unique feature is their potential to contribute to genetic diseases or evolutionary changes. While they can lead to harmful effects, such as cancer, they may also provide the raw materials for evolution, allowing species to adapt to new environments.
Insertions and Deletions
Insertions and deletions (often referred to as indels) are another important type of mutation. As the name implies, insertions involve the addition of one or more nucleotide bases, while deletions involve the removal of bases in the DNA sequence. These mutations can result in frameshift changes, altering the entire downstream protein sequence.
The key characteristic of insertions and deletions is the potential to cause significant changes in gene function and expression. This aspect makes them vital for understanding gene regulation and protein synthesis. They can lead to nonfunctional proteins or proteins with altered activity.
The unique feature of indels is their unpredictability. They can occur spontaneously or be induced by environmental factors. While they can cause genetic diseases, they may also lead to beneficial traits, contributing to genetic diversity.
DNA Repair Mechanisms
Nucleotide Excision Repair
Nucleotide excision repair is a critical mechanism for correcting DNA damage. This process is especially important in repairing bulky DNA adducts that distort the helical structure of DNA, such as those caused by UV radiation or chemical exposure.
A highlight of nucleotide excision repair is its ability to restore the DNA to its original state. The process involves identifying the damaged nucleotide, excising it, and filling in the gap with the correct nucleotides, using the complementary strand as a template.
This repair mechanism is beneficial in this article because it demonstrates a cellular defense against environmental insults. Its unique feature lies in repairing a broad range of DNA lesions, making it essential for the maintenance of genomic stability. However, deficiencies in this repair pathway can lead to increased mutation rates and cancer susceptibility.
Mismatch Repair
Mismatch repair is vital for correcting errors that occur during DNA replication. After DNA synthesis, the mismatch repair system identifies base pair mismatches that escape proofreading by DNA polymerases. This system is crucial in maintaining genome integrity.
A key characteristic of mismatch repair is its high fidelity in correcting replication errors. It targets mismatches and small insertions or deletions that may have occurred during DNA synthesis. This aspect makes it a popular topic for study in molecular biology.
The unique feature of mismatch repair is its ability to prevent the proliferation of mutations that arise during DNA replication. By ensuring accurate DNA replication, this mechanism helps maintain genetic stability. Nonetheless, failure of mismatch repair mechanisms can lead to hereditary cancer syndromes, emphasizing the importance of understanding this repair pathway.
Applications of DNA Research
Understanding DNA and its functions is fundamental in various fields of research and application. The importance of the applications of DNA research spans medicine, biotechnology, and forensic science. This section delves into two critical areas where DNA research has made significant impacts: genetic engineering and forensic science.
Genetic Engineering
Genetic engineering involves the direct manipulation of an organism's genes using biotechnology. This area of study opens numerous possibilities for advancements in medicine, agriculture, and industry. The key elements of genetic engineering include:
- Recombinant DNA Technology: This technique allows scientists to splice genes from one organism into another. For instance, the creation of insulin-producing bacteria has revolutionized diabetes treatment.
- CRISPR-Cas9: A groundbreaking technology that enables precise editing of the DNA sequence. It holds promise for treating genetic disorders by correcting mutations at specific sites within the genome.
- Transgenic Organisms: Organisms that have been genetically modified to express genes from another species. An example is Bt corn, which is engineered to resist pests, thus reducing the need for chemical pesticides.
The benefits of genetic engineering are immense. It has the potential to eliminate genetic diseases, increase food production, and develop new therapeutics. However, considerations regarding ethics, environmental impacts, and long-term effects must be taken into account to ensure responsible usage.
Forensic Science
Forensic science utilizes DNA analysis to solve crimes and identify individuals. DNA profiling has become a golden standard in criminal investigations and legal proceedings. Its significance lies in:
- Identification: DNA can link a suspect to a crime scene, establish paternity, or identify remains. Techniques such as Short Tandem Repeat (STR) analysis are commonly used for these purposes.
- Cold Cases: DNA testing can reopen unresolved cases by comparing old evidence with new DNA databases. This method has brought justice to many families who have waited years for answers.
- Exoneration: Advances in DNA analysis have led to the exoneration of wrongfully convicted individuals, highlighting the power of DNA evidence in the legal system.
The applications of DNA research in forensic science not only enhance the accuracy of investigations but also ensure that justice is served effectively. As technology evolves, the methods used for DNA analysis continue to become more sophisticated, leading to even greater applications in the future.
"DNA research is not just about understanding life at a molecular level; it also has profound implications for society, law, and ethics."
Finale
The conclusion serves a pivotal role in encapsulating the intricate themes and findings presented throughout the article. It synthesizes the comprehensive analysis of DNA's structure and function, aiming to reinforce the relevance of these insights within the broader realm of genetics and molecular biology. Understanding DNA is not merely an academic exercise; it underpins significant advances in diverse fields such as medicine, forensics, and biotechnology. This allows researchers and professionals to harness the power of genetic information for practical applications.
Summarizing Key Points
In the previous sections, we explored several essential components that define DNA:
- Nucleotides: The building blocks, comprising adenine, guanine, cytosine, and thymine.
- Double Helix Structure: Fundamental to DNA's stability and function, including the significance of major and minor grooves.
- Base Pairing Rules: Determining how genetic information is effectively stored and transmitted.
- Replication Mechanisms: Essential processes that ensure accurate genetic transmission.
- Applications of DNA Research: Various fields that benefit from understanding DNA, including genetic engineering and forensic science.
This summary highlights how these components are interconnected, showcasing their collective importance in understanding the genomic landscape.
Future Directions in DNA Research
Looking ahead, the trajectory of DNA research holds immense potential. Some areas for exploration include:
- CRISPR Technology: Advancements in gene editing that could revolutionize how we treat genetic disorders.
- Synthetic Biology: Developing new biological parts and systems to create sustainable solutions to modern problems.
- Personalized Medicine: Tailoring treatment plans based on individual genetic profiles, enhancing efficacy and outcomes.
- Environmental DNA (eDNA) Monitoring: Utilizing DNA to assess biodiversity and ecosystem health, enabling better conservation strategies.
The continuous evolution of DNA research promises not only to deepen our understanding of life at its most fundamental level but also to address pivotal global challenges. It is essential that educators, scholars, and practitioners engage in this ongoing dialogue to determine the ethical implications and societal impacts as we advance further into the realm of genetic discovery.