Critical Role of DNase Treatment After RNA Extraction
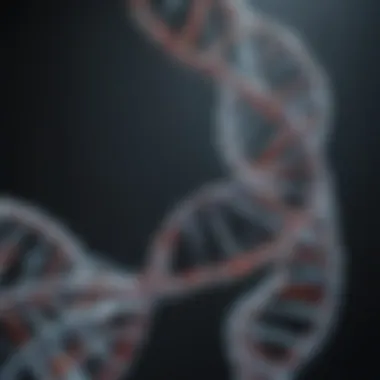
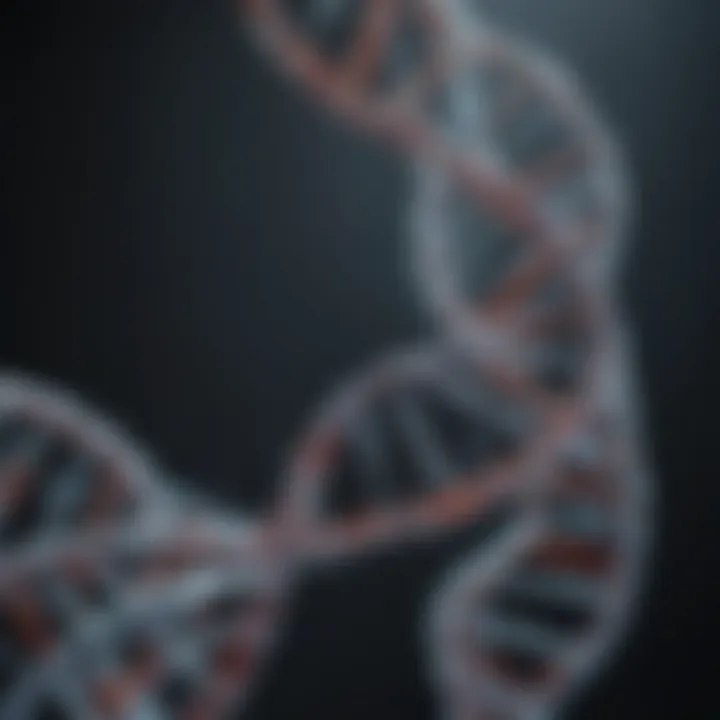
Intro
The separation of ribonucleic acid (RNA) from a sample is a pivotal step in molecular biology research. However, a successful extraction is only the beginning. One critical aspect that often follows is treatment with deoxyribonuclease (DNase). This step is essential for researchers striving to achieve high-quality RNA by eliminating DNA contaminants. The intricate dynamics between RNA and DNA require a nuanced approach to ensure the integrity and purity of the RNA obtained.
This article delves into the significance of DNase treatment after RNA extraction, providing insights into its mechanisms, methodologies, and implications. In an era where accuracy in RNA analysis is paramount, understanding the nuances of this process becomes crucial. Let's unravel the complexities surrounding this topic.
Research Overview
Key Findings
When examining DNase treatment, several noteworthy observations emerge:
- Purity Boost: Effective DNase treatment significantly enhances RNA purity, reducing the chance of DNA interference during downstream applications.
- Mechanistic Insights: DNase enzymes operate primarily by hydrolyzing the phosphodiester bonds within the DNA, ultimately leading to its degradation into smaller fragments.
- Protocol Optimization: Not all DNase treatments are equal; optimizing conditions such as enzyme concentration and incubation time is crucial for effective DNA degradation.
These findings collectively underscore the indelible importance of DNase treatment in RNA studies, especially for applications where precision is essential, like qPCR or RNA-seq.
Study Methodology
A variety of methodologies are employed when investigating DNase treatment's effectiveness. Research typically involves a side-by-side comparison of RNA samples processed with and without DNase treatment to ascertain the impact on quality and integrity. By applying various concentrations of DNase and observing the degradation of contaminating DNA, researchers can establish optimal conditions that yield the highest RNA purity. Techniques involving gel electrophoresis and spectrophotometric analysis commonly accompany these studies for quantification and quality assessment, ensuring robust data collection.
Background and Context
Historical Background
The journey into RNA extraction and cleanup has traversed several decades. Initially, conventional methods lacked precision, resulting in RNA samples plagued by contaminants, primarily DNA. The introduction of DNase treatment marked a turning point in molecular biology, shifting paradigms towards cleaner and more reliable RNA studies. Scientists have since developed a range of DNase enzymes, each tuned to different applications and types of samples.
Current Trends in the Field
Preamble to RNA Extraction
The extraction of RNA is a pivotal step in various areas of molecular biology, genomics, and biotechnology. It serves as the gateway for understanding cellular functions, gene expression, and even the mechanisms of disease. The success of downstream applications largely hinges on the quality and integrity of the RNA extracted. If the RNA is muddied by contaminants, it can significantly skew results, leading researchers down a path filled with potential misinterpretations.
Fundamentals of RNA Isolation
At its core, RNA isolation involves several vital stages: cell lysis, separation of RNA from other biomolecules, and stabilization of the isolated RNA. Each stage carries its own challenges yet offers unique opportunities for optimization. For instance, during the cell lysis phase, one must carefully choose the lysis buffer. The right buffer can make or break the yield and quality of the RNA. Using too harsh a method can degrade RNA, while a milder approach might not liberate enough RNA from the cells.
Moreover, keeping the process efficient and preventing RNA degradation is critical. This highlights the delicate balance required during RNA extraction. Contaminants, particularly genomic DNA, can persist through extraction, complicating results during analysis. That's where DNase treatment comes into play to ensure that DNA is effectively removed while maintaining the RNA's integrity.
Importance of RNA Quality
Ensuring high-quality RNA is indispensable for the reliability of experimental outcomes. Poor-quality RNA can lead to ambiguous data, wasted resources, and time-consuming troubleshooting. High-quality RNA exhibits high purity — often denoted with specific A260/A280 ratios — and is free from degradation. The presence of intact ribosomal RNA, for instance, is a strong indicator of overall RNA quality.
Failure to consider RNA quality during the extraction process can have ripple effects.
Common downstream applications — such as quantitative PCR or RNA sequencing — rely heavily on the integrity of the material used.
"If the foundation isn't solid, the structure built on it will always be shaky."
Therefore, it becomes essential to not only extract RNA but to do so under conditions that optimize yield and purity, making subsequent DNase treatment necessary to ensure DNA contaminants are eradicated.
In summary, RNA extraction is far from a trivial task. It requires meticulous attention to detail to ensure that the RNA obtained is of the highest quality, free from contaminants to allow for accurate and reproducible results. By understanding the fundamentals and emphasizing quality, researchers can place themselves on a steadier path towards insightful discoveries and innovations.
Challenges in RNA Purification
In the pursuit of pristine RNA, researchers often grapple with challenges during purification. These hurdles can significantly impact the quality and usability of RNA in various downstream applications. Addressing these challenges is fundamental not only to achieving clear experimental outcomes but also to maintaining the integrity of biological analysis. A deeper understanding of the issues at hand helps streamline protocols and avoid pitfalls that might skew results or render samples unusable.
Contamination by Genomic DNA
One of the primary obstacles in RNA purification is contamination by genomic DNA. Genomic DNA can co-purify with RNA during extraction, creating a mixed sample that complicates further analysis and results interpretation. In most cases, genomic DNA presence can lead to false positives in quantitative PCR or RNA sequencing results.
To make matters worse, the mere presence of this DNA can interfere with enzyme activity, especially in reactions that are specific to RNA, such as reverse transcription. One way to approach this issue is through careful selection of extraction kits and protocols that emphasize the separation of RNA from DNA. Additionally, using DNase treatment post-extraction serves as a robust method to eliminate any residual DNA. It’s crucial for researchers to be aware of potential contamination sources and take preventive measures during the initial stages of extraction and purification.
Impact on Experimental Results
The impact of genomic DNA contamination extends beyond just the purity of RNA; it can ripple through the entire research process. From varying expression levels during qPCR assays to erroneous data in high-throughput sequencing, the repercussions of this contamination can mislead even the most seasoned researchers. For instance, detectable levels of DNA can artificially elevate RNA quantity readings, suggesting that a sample has a higher RNA concentration than it actually does.
To mitigate these issues, researchers must prioritize quality control measures. Regular verification of RNA samples, such as using spectrophotometry or gel electrophoresis, helps assess the purity and integrity of the isolated RNA. However, the significance of addressing DNA contamination goes beyond mere sample integrity. It shapes the overall scientific narrative created from the data.
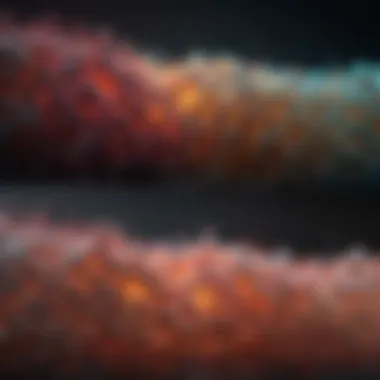
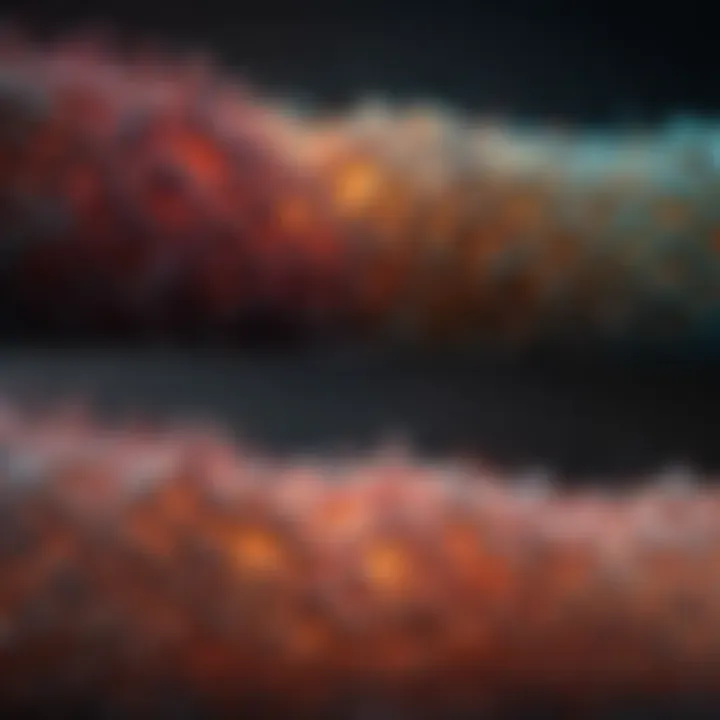
"High-quality RNA is the backbone of reliable findings in molecular research. A single oversight in purification can taint the broader outcomes of a study."
Moreover, understanding the specific downstream applications can drive tailored solutions. For example, in applications requiring high sensitivity, such as single-cell RNA sequencing, the purity of RNA becomes an essential aspect that cannot be compromised. Knowing how genomic DNA can skew results prompts researchers to adopt rigorous protocols and make informed decisions in their experimental designs.
Overview of DNase Treatment
Understanding DNase treatment is pivotal in the realm of molecular biology, especially after RNA extraction. While obtaining RNA free from contaminants is crucial, what follows extraction is just as important—this is where DNase treatment comes into play. This section delves into the significance of effectively eliminating DNA residues. Such removal ensures that the integrity of RNA remains intact for accurate analyses, which can significantly alter the outcomes of experiments, especially in sensitive techniques like qPCR and RNA sequencing.
What is DNase?
Deoxyribonuclease, often dubbed DNase, refers to a family of enzymes that specialize in the breakdown of DNA. The role of DNase in molecular biology hinges on its ability to cleave the phosphodiester bonds within the DNA backbone. Importantly, this enzymatic action is vital during RNA work because it prevents DNA from co-purifying with RNA, which can lead to skewed experimental results.
Concretely, DNases can be categorized into two main types: DNase I and DNase II. DNase I, sourced from bovine pancreas, operates optimally in a magnesium-dependent manner and is renowned for digesting both double-stranded and single-stranded DNA. On the other hand, DNase II, typically found in various tissues, functions well in acidic conditions and predominantly targets double-stranded DNA. This distinction highlights the diverse applications of DNases depending on the specific requirements of RNA work.
Mechanism of Action
The mechanism through which DNase functions is quite straightforward yet fascinating. Upon introduction into a solution containing DNA, DNase enzymes hydrolyze the DNA strands, resulting in a collection of smaller oligonucleotides. This breakdown occurs through the enzymatic catalysis of the phosphodiester bonds, which forks the DNA into nucleotides and smaller fragments. Such fragmentation effectively neutralizes the DNA that could interfere with subsequent RNA analyses.
It's key to ensure that the conditions for DNase action – including pH, temperature, and ionic strength – are optimized. Typically, a neutral to slightly alkaline pH enhances the effectiveness of DNase, making the enzymatic action smoother and more complete. As a rule of thumb, if treatment is not executed with careful consideration of these factors, researchers might end up with residual DNA in their RNA preparations, thereby compromising the integrity of all downstream applications.
"Effective DNase treatment can essentially safeguard the quality of RNA, impacting everything from gene expression studies to therapeutic development."
In summary, integrating DNase treatment into RNA extraction processes is indispensable for achieving high-quality RNA devoid of DNA contamination. It opens the door for more reliable and reproducible results in molecular biology, enabling researchers to proceed with confidence in their findings.
Types of DNase Enzymes
In the realm of molecular biology, understanding the nuances of DNase enzymes is paramount when it comes to enhancing RNA retrieval processes. Not all DNases are created equal, and the efficiency of removing DNA can vary significantly depending on the type employed. Here, we will delve into the two primary DNase enzymes used frequently in laboratory protocols: DNase I and DNase II, closely examining their individual characteristics, benefits, and optimal usage scenarios.
DNase
DNase I, often regarded as the go-to enzyme for routine applications, plays a pivotal role in degrading extracellular DNA. This enzyme is particularly effective when addressing genomic DNA contaminations in RNA samples. DNase I operates optimally at neutral pH, making it compatible with various buffer systems commonly used in RNA work. The enzyme is sensitive to different ionic strengths; hence, for successful digestion, maintaining the right salt concentration is crucial.
One notable feature of DNase I is its ability to cleave DNA in a nonspecific manner, thereby producing short oligonucleotides. This can be a double-edged sword; while it effectively reduces unwanted DNA, it can inadvertently lead to RNA degradation if the treatment is not carefully monitored. Thus, one must adhere to specific conditions during treatment, such as temperature and timing, to limit any detrimental effects on RNA integrity while ensuring thorough digestion of DNA.
Benefits of DNase I:
- Efficiently degrades genomic DNA, enhancing RNA purity.
- Works well within physiological conditions, broadening its applicability across various experiments.
- Can be easily removed post-treatment using standard purification methods.
DNase
In contrast to DNase I, DNase II is classified as an endonuclease that functions optimally in acidic environments. Initially isolated from the pancreatic tissues of animals, this enzyme not only exhibits a different activity profile but also a unique role in cellular processes, including apoptosis and autophagy. Although less commonly used than DNase I for nucleic acid manipulation, its ability to degrade DNA in acidic conditions offers certain advantages, particularly in specific experimental setups where manipulating the pH can facilitate desired outcomes.
One significant advantage of DNase II is its propensity for targeted degradation of DNA resulting from cellular debris, making it quite useful in scenarios where residual DNA must be removed without compromising RNA samples. Its activity, however, can also be affected by various factors such as the specific buffer used and temperature settings, necessitating careful optimization. Moreover, researchers should take into account that DNase II enzymes are more complex in terms of availability and characterization compared to DNase I.
Benefits of DNase II:
- Effective in environments where pH is not neutral, enabling versatility in experimental design.
- Assists in the cleanup of samples by efficiently degrading cell-free DNA without harming RNA.
"Choosing the right DNase enzyme can mean the difference between a successful RNA experiment and one fraught with contamination issues."
When selecting a DNase enzyme for RNA extraction protocols, it’s critical to consider the specific requirements of your study, the nature of the RNA, and the potential interactions with the reagents used. Knowledge of the strengths and limitations of both DNase I and DNase II equips researchers to make informed decisions that can significantly impact the quality and integrity of RNA samples in downstream applications.
Protocol for DNase Treatment
In the realm of molecular biology, establishing a robust protocol for DNase treatment is essential for yielding RNA samples that are not only pure but also reliable for downstream applications. The success of RNA extraction hinges significantly on how well one navigates the complexities involved in DNA removal. Following up RNA extraction with DNase treatment adds a layer of assurance that genomic DNA contaminants are mitigated, an aspect that cannot be overlooked if erroneous data in experiments is to be avoided.
Preparation of Reagents
Before diving headfirst into the step-by-step procedure, it's crucial to gather all necessary reagents cautiously. Precision is the name of the game here as preparation can mean the difference between success and failure in DNase treatment.
- DNase Solution: Select the appropriate DNase enzyme, often DNase I is favored due to its effectiveness. Reconstitute it following the manufacturer's guidelines.
- Buffer Solutions: Prepare the appropriate buffer, generally a Tris buffer at pH 7.5, which helps to maintain the enzyme’s activity.
- Inhibitors and Additives: Sometimes, including stabilizers such as calcium or magnesium ions may enhance DNase activity but note that these can also inhibit the downstream applications if not carefully removed afterward.
Proper handling and storage of reagents prior to use are equally significant; they should be kept at appropriate temperatures (often -20°C for DNase) to maintain their effectiveness.
Step-by-Step Procedure
Now, let’s outline a practical approach to execute the DNase treatment. Each step is interconnected, and skipping one could lead to lingering DNA, which would compromise data integrity. Here’s how to go about it:
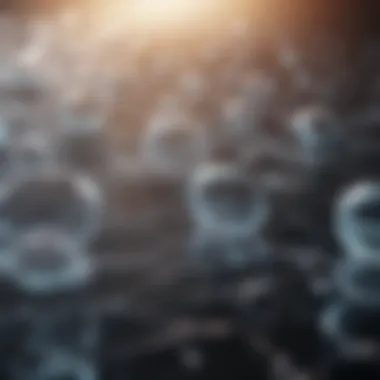
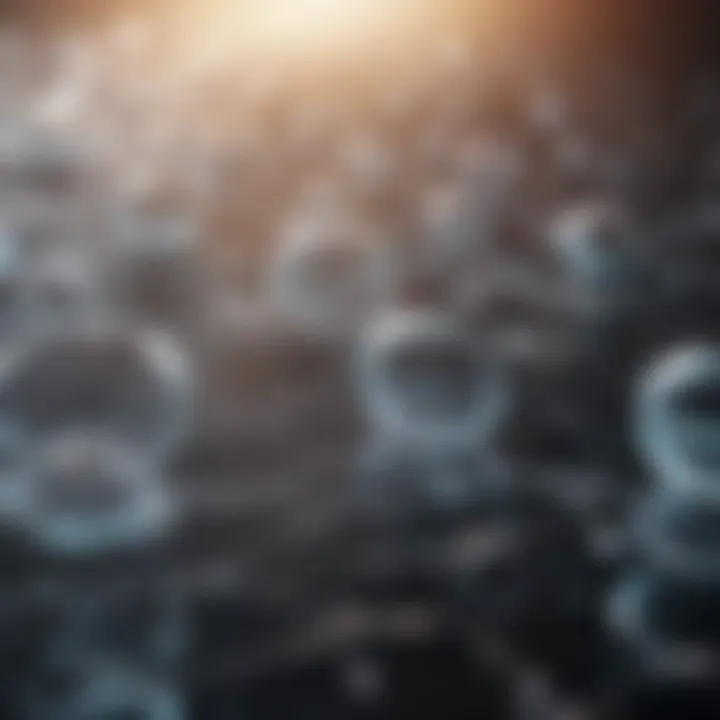
- Sample Preparation: Start with your RNA sample, ensuring it’s free of any organic solvents and fully thawed.
- Combine Reagents: In a clean tube, mix your RNA sample with the prepared DNase solution and the buffer to achieve the optimal concentration.
- Incubation: Place the tube in an incubator or water bath, typically around 37°C, for a duration of 20 minutes. This allows the DNase to act on the DNA.
- Stop Reaction: After incubation, inactivate the DNase by either adding a stop solution, usually EDTA, which chelates the metal ions required for DNase function or by heating the mixture to 65°C for 10 minutes. This is crucial as residual enzymatic activity post-treatment can degrade your precious RNA.
- Purification: Finally, purify the mixture using silica-based column methods or phenol-chloroform extraction to ensure any residual DNase and degradation products are removed.
Achieving a high level of precision during the protocol ensures a smooth road ahead for your RNA analysis.
Post-Treatment Clean-Up
Once the DNase treatment is complete, post-treatment clean-up becomes the next focal point. This is where your expertise in handling RNA will shine through. The clean-up process serves not only to eliminate leftover reagents but also to ensure the RNA is in prime condition for downstream applications such as quantitative PCR or RNA sequencing.
- Silica Membrane Columns: These are effective for removing any contaminants. Simply apply your treated sample to the column and follow the recommended washing steps.
- Ethanol Precipitation: Alternatively, one can use ethanol precipitation to concentrate the RNA and wash away impurities. This involves mixing your RNA with a 0.3 M sodium acetate solution and two volumes of cold ethanol, followed by an overnight -80°C incubation before centrifugation.
- Quality Assessment: Finally, assess the integrity and purity of the RNA using spectrophotometry or gel electrophoresis. This quality check is not just a formality; it can illuminate any potential issues that could affect experimental outcomes.
By adhering to a meticulously structured protocol for DNase treatment, researchers not only enhance the reliability of their RNA analysis but also significantly contribute to the overall understanding of molecular interactions in biological systems.
Considerations During DNase Treatment
When delving into DNase treatment after RNA extraction, there are a number of critical factors to bear in mind. These considerations ensure not only the efficacy of the DNase treatment but also the overall success of downstream applications involving RNA. The proper execution of each of these elements can profoundly affect RNA integrity and quality.
Temperature and Time Optimization
Temperature and time are two of the fundamental elements that can dramatically influence the effectiveness of DNase treatment.
- Temperature Effects: DNase enzymes possess specific temperature ranges where they perform optimally. Generally, for most DNase I preparations, temperatures around 37°C are preferred. However, if the temperature strays too far from this optimal point, the enzyme activity may diminish significantly. This reduction can lead to incomplete digestion of DNA, leaving unwanted genomic DNA in the RNA sample, which can hinder later experiments, like qPCR or sequencing. Conversely, excessively high temperatures can also denature the enzyme, rendering it ineffective at removing DNA.
- Timing: The duration of DNase treatment varies based on several factors, including the concentration of the enzyme, the amount of DNA present, and the nature of the sample. Typically, incubation times of 15-30 minutes at optimal temperature are recommended. But if you find it’s taking longer, consider that over-treating RNA with DNase can lead to its degradation. Therefore, a careful balance of time and conditions is vital. Monitoring these parameters closely enables you to maximize the efficiency of the clearing process.
To put it succinctly:
- Stick to optimal temperature ranges, and
- Adjust incubation times wisely to maintain RNA integrity.
pH Conditions
Another aspect often overlooked is the pH during DNase treatment. The enzyme DNase is sensitive to pH levels, which can dramatically impact its efficacy and the subsequent quality of RNA.
- Optimal pH Levels: Most DNase enzymes operate best around a neutral pH of approximately 7.0 to 8.0. This pH range ensures that the enzyme remains active and can effectively cleave phosphodiester bonds in DNA. If the pH strays towards either the acidic or basic side, the activity of the DNase may wane. Using buffers like Tris-HCl or phosphate can help maintain the necessary conditions during the digestion process.
- Buffer Selection: Additionally, choosing the right buffer can influence the enzyme's stability. Not every buffer is suitable for every enzymatic reaction. Understanding how your chosen buffer interacts with the enzyme at different pH levels is crucial.
Here’s a quick rundown of pH considerations:
- Use neutral pH buffers to maintain enzymatic activity.
- Ensure periodic checking of pH levels during the treatment process.
Proper management of temperature, time, and pH during DNase treatment can mean the difference between success and potential failure in your RNA experiments.
By understanding these key factors, researchers can improve the precision of their RNA analyses and maintain the integrity of samples across various downstream applications.
Downstream Applications of RNA
The importance of RNA in biological research cannot be overstated. Following DNase treatment, the integration of RNA into various applications becomes pivotal. The processes that rely on purified RNA are diverse, ranging from gene expression analysis to diagnostics. In this section, we'll delve into two significant downstream applications of RNA: Quantitative PCR and RNA sequencing.
Quantitative PCR
Quantitative PCR (qPCR), also known as real-time PCR, has emerged as a cornerstone technique in molecular biology. This method enables researchers to quantify RNA levels, providing insight into gene expression profiles. The accuracy of qPCR is highly dependent on the quality of RNA used in the reaction. Contaminants such as residual genomic DNA can skew the results, leading to misinterpretation of gene expression levels.
In essence, qPCR amplifies specific RNA sequences while measuring the quantity of amplified product as the reaction progresses. To perform qPCR successfully, the following considerations are crucial:
- RNA Integrity: High-quality, intact RNA is essential for reliable results. The presence of degraded RNA can lead to inefficient amplification.
- DNase Treatment: Effective DNase treatment minimizes DNA contamination, ensuring that the amplification is specific to RNA. This makes the DNase step not just beneficial, but practically indispensable.
- Comparative Analysis: qPCR enables comparison of gene expression levels across different conditions or treatments, aiding in the understanding of underlying biological processes.
When carefully executed, qPCR provides quantifiable data that can be instrumental in clinical diagnostics, fundamental research, and even therapeutic monitoring.
RNA Sequencing
RNA sequencing (RNA-seq) has transformed our understanding of the transcriptome. Unlike qPCR, which typically measures a limited number of targets, RNA-seq offers a comprehensive view of the entire RNA content in a sample. It captures not only the presence of transcripts but also their structure and variation. However, the success of RNA-seq is contingent upon high-quality RNA free from DNA contamination.
Considerations for successful RNA sequencing include:
- Sample Preparation: Proper sample handling during extraction and DNase treatment guarantees the integrity of RNA.
- Library Construction: Construction of sequencing libraries requires pure RNA to avoid bias in fragment selection, which could occur due to DNA carryover.
- Data Interpretation: With vast data generated, accurate interpretation hinges on the quality of the RNA used. Poor-quality RNA can lead to misleading results in transcript quantification and annotation.
RNA-seq applications range from studying gene expression to discovering novel non-coding RNAs. As technology evolves, the insights gleaned from these sequencing analyses continue to impact fields such as personalized medicine and drug development.
In summary: The downstream applications of RNA demand a meticulous approach to ensure data integrity. By focusing on RNA quality through effective DNase treatment, researchers lay a solid foundation for further exploration and discovery in the molecular biology landscape.
Common Issues and Solutions
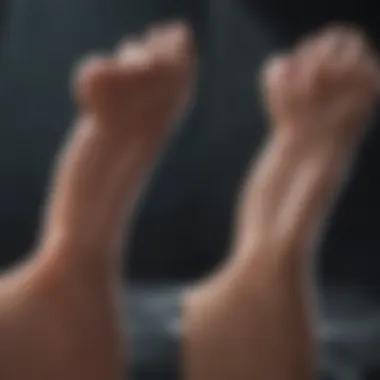
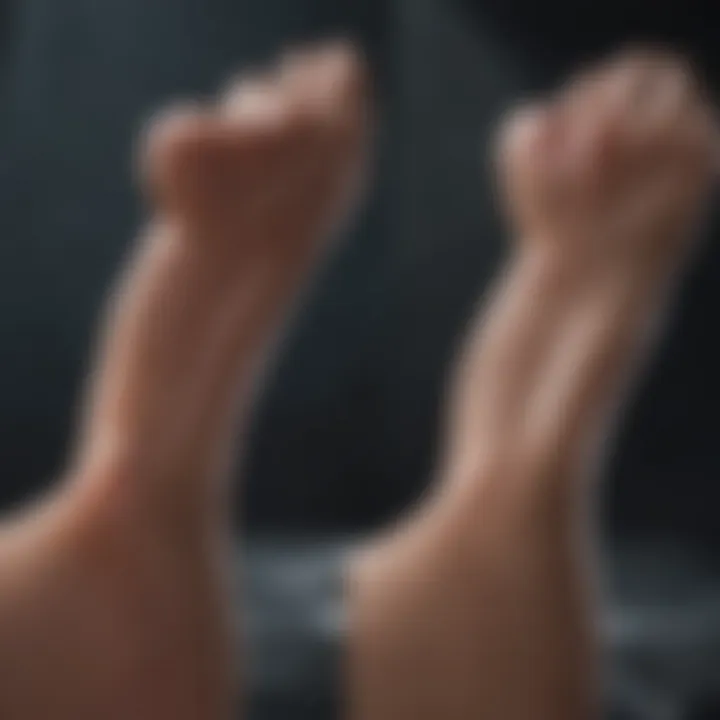
Addressing common issues that arise in DNase treatment is essential for achieving the desired purity and quality of RNA samples. Unsuccessful DNase treatment may lead to undesired outcomes in downstream applications, affecting the reliability of experimental results. By tackling these issues head-on, researchers can significantly improve the integrity of their RNA analyses. Understanding these challenges not only aids in refining techniques but also equips experimenters with strategies to enhance productivity and accuracy in their molecular biology work.
Incomplete DNase Digestion
Incomplete digestion during DNase treatment is a frequent hurdle that many researchers encounter. When the DNase enzyme does not fully degrade the genomic DNA contaminants, the sample can remain tainted, leading to inaccuracies in subsequent analyses. For example, in quantitative PCR, which relies heavily on the specificity of RNA, traces of DNA can skew quantitative results, leading researchers down the wrong path.
To mitigate this issue, it is crucial to optimize several factors:
- Enzyme Concentration: Using the right amount of DNase is vital. Too little might not effectively degrade all the DNA, while too much can lead to unwanted RNA degradation.
- Reaction Time: Allow sufficient time for the reaction to occur. Shortening the digestion time may not give the DNase enough opportunity to act thoroughly.
- Temperature Conditions: Ensuring the reaction is carried out within an optimal temperature range can also enhance the efficacy of the digestion process. DNases often work best at specific temperatures, and deviations can impact activity.
Ensuring complete digestion not only preserves the quality of RNA but also boosts confidence in the reproducibility of your results.
RNA Degradation Risks
Another pressing concern during DNase treatment is the potential for RNA degradation. While DNases specifically target DNA, improper handling or conditions can lead to unintended damage to RNA. Such degradation can compromise the quality of the RNA, impacting experimental results and interpretations.
Here are several considerations to reduce RNA degradation risks during DNase treatment:
- Use of RNase-Free Equipment: To prevent contamination, always work with RNase-free tubes, pipette tips, and other materials. This is a fundamental precaution since RNases are notoriously stable and can wreak havoc on your precious RNA samples.
- Proper Buffer Conditions: The choice of buffers can influence both DNase activity and RNA stability. For instance, maintaining the correct pH is essential as extreme pH levels can lead to RNA hydrolysis by endogenous enzymes.
- Monitoring Reaction Duration: Overly prolonged reactions can inadvertently set the stage for RNA degradation. Keep an eye on the clock and ensure you're following established protocols for timing.
"RNA quality is the backbone of trustworthy molecular biology. Address common issues, and you'll pave the way for success in your studies."
By proactively addressing these concerns, you can ensure that your RNA analysis remains robust and reliable, ultimately enhancing the quality of your scientific inquiries.
Comparative Studies on DNase Treatment Methodologies
Understanding the nuances of DNase treatment methodologies is central to advancing RNA extraction techniques. Each method possesses distinct advantages and limitations that can significantly influence the purity and quality of RNA. Researchers often find themselves faced with a multitude of choices when it comes to DNase applications. This section aims to critically evaluate both enzymatic and chemical methods of DNase treatment, providing insights into their efficiencies and suitability for specific applications.
Enzymatic Versus Chemical Methods
When it comes to DNase treatment, two primary approaches emerge: the use of enzymatic treatments versus chemical alternatives. Enzymatic methods, typically involving DNase I or II, go for a more biological approach to degrading DNA contaminants. They work by hydrolyzing the phosphodiester bonds in the DNA backbone, leading to the release of nucleotides.
On the other hand, chemical methods often employ agents like sodium hydroxide or other alkalis to achieve similar ends. For some experimental setups, chemical methods can be more straightforward and quicker, especially when large volumes of samples are at play or when conditions are too extreme for enzymes to function well.
Both approaches have their pros and cons:
- Enzymatic Advantages:
- Chemical Advantages:
- Highly specific to nucleic acids, minimizing damage to RNA.
- Typically have milder reaction conditions, preserving RNA integrity.
- Often faster in terms of procedure time.
- Can be more cost-effective when working with larger batches of samples.
However, enzymatic treatments generally yield better quality RNA for downstream applications like quantitative PCR and RNA sequencing since they are less likely to introduce additional contaminants. Each method requires careful consideration of the experimental goals, and often, a hybrid approach may offer the best of both worlds.
Efficiency of Different DNases
The performance of DNases can vary significantly based on their source and characteristics. DNase I, derived from bovine pancreas, is the most commonly used form in labs. Its ability to efficiently digest DNA in a variety of conditions is well-documented. However, DNase I can be sensitive to certain buffers and temperature variations, necessitating precise control during the experimental process.
Conversely, DNase II, sourced from human tissues, operates optimally at acidic pH levels and is known for its robustness against conditions that might inactivate DNase I.
Efficiency can also depend on several factors:
- Temperature: Elevated temperatures often improve the activity of DNases but may jeopardize RNA stability if not monitored vigilantly.
- Concentration: The amount of DNase used must be tailored to the quantity of target DNA present. Ideally, titration experiments should be employed to find the optimal concentration.
- Incubation Time: Longer treatment times can lead to more thorough degradation of DNA, but there's always a risk of over-digestion, potentially harming the RNA.
In comparative studies, it's imperative to evaluate how the choice of DNase affects RNA recovery quantitatively and qualitatively. Various studies have shown differences in RNA yields and qualities with different DNA removal methodologies, which ultimately impact the result of subsequent analyses.
"The choice of DNase should align with the specific needs of your research. Underestimating the differentiated effects can lead to flawed experimental outcomes"
Future Directions in RNA Research
The field of RNA research has evolved rapidly, driven by technological advancements and a growing appreciation for the roles of RNA beyond mere intermediaries in protein synthesis. The importance of identifying future directions in RNA research is crystal clear, especially in the wake of recent innovations. As researchers push the boundaries of what we understand about RNA, it becomes essential to harness novel methodologies and tackle emerging challenges.
One critical element in advancing RNA research is enhancing DNase technology. As discussed earlier, DNase treatment is pivotal for purifying RNA from genomic DNA contaminants. Future research may delve into the development of specific DNase variants or enhanced enzyme formulations that ensure more complete removal of DNA without degrading RNA integrity. Such advancements could significantly improve downstream applications in areas like therapeutics and diagnostics. Moreover, advances in automation and high-throughput screening methods could propel the accessibility of DNase treatments, making them more widely applicable across laboratories.
Advancements in DNase Technology
The next wave of innovation in DNase technology might include the creation of targeted DNases designed to selectively degrade specific forms of DNA. For instance, engineered DNases might focus on unwanted viral DNA in clinical samples, critical for further studies on viral infections and their effects on RNA. Additionally, real-time monitoring of DNase activity could become a significant research area. Techniques such as fluorescence-assisted detection could allow researchers to visualize and quantify DNase action dynamically, thereby optimizing their protocols in real time.
"The future holds exciting possibilities for refining DNase treatment protocols that can yield even purer samples, critical for high-stakes research."
Innovative RNA Handling Techniques
The way RNA is handled in laboratories is equally vital to the outcomes of research. Moving forward, innovative RNA handling techniques will likely emerge, driven by a desire to minimize degradation and contamination. For example, the use of encapsulated or modified-reaction vessels that are engineered to inhibit RNase activity could transform current practices. With these, researchers can ensure that their RNA remains uncontaminated during extraction and subsequent procedures.
Furthermore, the integration of machine learning and artificial intelligence into RNA research workflows has the potential to revolutionize how RNA integrity is assessed. Algorithms trained on large data sets can predict optimal conditions for RNA handling and storage. This predictive approach may enable labs to tailor their methods to specific RNA samples, ensuring that they maintain their quality throughout the research process. As the landscape of RNA research continues to shift, researchers must remain adaptable, embracing these advancements to push their boundaries and glean new insights.